Abstract
Background
Increased radioresistance due to previous irradiation or radiosensitivity due to human papilloma virus (HPV) infection can be observed in head and neck squamous cell carcinoma (HNSCC). The DNA-damage response of cells after exposure to DNA-damaging agents plays a crucial role in determining the fate of exposed cells. Tightly regulated and interconnected signaling networks are activated to detect, signal the presence of and repair the DNA damage. Novel therapies targeting the DNA-damage response are emerging; however, an improved understanding of the complex signaling networks involved in tumor radioresistance and radiosensitivity is needed.Materials and methods
In this study, we exposed isogenic human HNSCC cell lines with altered radiosensitivity to DNA-damaging agents: radiation, cisplatin and bleomycin. We investigated transcriptional alterations in the DNA-damage response by using a pathway-focused panel and reverse-transcription quantitative PCR.Results
In general, the isogenic cell lines with altered radiosensitivity significantly differed from one another in the expression of genes involved in the DNA-damage response. The radiosensitive (HPV-positive) cells showed overall decreases in the expression levels of the studied genes. In parental cells, upregulation of DNA-damage signaling and repair genes was observed following exposure to DNA-damaging agents, especially radiation. In contrast, radioresistant cells exhibited a distinct pattern of gene downregulation after exposure to cisplatin, whereas the levels in parental cells were unchanged. Exposure of radioresistant cells to bleomycin did not significantly affect the expression of DNA-damage signaling and repair genes.Conclusions
Our analysis identified several possible targets: NBN, XRCC3, ATR, GADD45A and XPA. These putative targets should be studied and potentially exploited for sensibilization to ionizing radiation and/or cisplatin in HNSCC. The use of predesigned panels of DNA-damage signaling and repair genes proved to offer a convenient and quick approach to identify possible therapeutic targets.Free full text

Expression of DNA-damage Response and Repair Genes After Exposure To DNA-damaging Agents in Isogenic Head and Neck Cells with Altered Radiosensitivity
Abstract
Background
Increased radioresistance due to previous irradiation or radiosensitivity due to human papilloma virus (HPV) infection can be observed in head and neck squamous cell carcinoma (HNSCC). The DNA-damage response of cells after exposure to DNA-damaging agents plays a crucial role in determining the fate of exposed cells. Tightly regulated and interconnected signaling networks are activated to detect, signal the presence of and repair the DNA damage. Novel therapies targeting the DNA-damage response are emerging; however, an improved understanding of the complex signaling networks involved in tumor radioresistance and radiosensitivity is needed.
Materials and methods
In this study, we exposed isogenic human HNSCC cell lines with altered radiosensitivity to DNA-damaging agents: radiation, cisplatin and bleomycin. We investigated transcriptional alterations in the DNA-damage response by using a pathway-focused panel and reverse-transcription quantitative PCR.
Results
In general, the isogenic cell lines with altered radiosensitivity significantly differed from one another in the expression of genes involved in the DNA-damage response. The radiosensitive (HPV-positive) cells showed overall decreases in the expression levels of the studied genes. In parental cells, upregulation of DNA-damage signaling and repair genes was observed following exposure to DNA-damaging agents, especially radiation. In contrast, radioresistant cells exhibited a distinct pattern of gene downregulation after exposure to cisplatin, whereas the levels in parental cells were unchanged. Exposure of radioresistant cells to bleomycin did not significantly affect the expression of DNA-damage signaling and repair genes.
Conclusions
Our analysis identified several possible targets: NBN, XRCC3, ATR, GADD45A and XPA. These putative targets should be studied and potentially exploited for sensibilization to ionizing radiation and/or cisplatin in HNSCC. The use of predesigned panels of DNA-damage signaling and repair genes proved to offer a convenient and quick approach to identify possible therapeutic targets.
Introduction
The DNA-damage response (DDR) plays a crucial role in determining the fate of cells after exposure to DNA-damaging agents. DNA-damage sensors, transducers and effectors are involved in tightly regulated and interconnected pathways.1 In the efficient response to DNA damage, the detection of defects in DNA structure is followed by cell cycle arrest and the activation of appropriate repair mechanisms via the activation of various DNA-repair pathways. In the event of excessive or irreparable DNA damage, cell cycle arrest eventually leads to the elimination of cells with such damage through mechanisms such as apoptosis or mitotic catastrophe. In addition, permanent cell cycle arrest in the form of senescence can be induced.2
The main types of radiation-induced DNA damage include base damage, single-strand breaks and double-strand breaks.3 Cisplatin (CDDP) is one of the most commonly used anticancer drugs and binds to sequence-specific DNA sites, forming intrastrand DNA adducts and interstrand DNA crosslinks.4, 5, 6 Similar to radiation, bleomycin (BLEO) induces both single-strand and double-strand DNA breaks.7,8 Spontaneous intracellular activation of both CDDP and BLEO produces reactive oxygen species (ROS), which facilitate further oxidative damage to DNA.5,8 Depending on the complexity of DNA damage, different repair pathways are activated through the DNA-damage signaling cascade.1 The ability of tumor cells to induce an effective DNA-damage response immediately after exposure to DNA-damaging agents promotes radio- and chemoresistance. On the other hand, failure to sufficiently activate DNA repair can lead to tumor cell death.
Both increased radioresistance and radiosensitivity can be observed in head and neck squamous cell carcinoma (HNSCC). Radioresistant HNSCC is prone to recur in previously irradiated areas from the surviving radioresistant cells.9,10 On the other side of the radiosensitivity spectrum of HNSCC, encouragingly favorable survival outcomes in human papillomavirus (HPV)-induced oropharyngeal SCCs to the standard combination of concurrent combination of irradiation (IR) with CDDP encourage thinking about modifying therapy intensity.11,12 Indeed, in the last decade, several de-escalation therapeutic approaches have been proposed for the treatment of HPV-positive tumors due to their increased radiosensitivity to reduce treatment-associated toxicities while maintaining therapeutic efficacy.13, 14, 15 However, novel and effective therapies as alternatives to IR and CDDP are needed to treat recurrent resistant tumors. BLEO-based therapies are interesting alternatives for the treatment of HNSCC because BLEO, although considered a radiomimetic drug, differs from radiation in the way it induces damage.16
Improved therapeutic outcomes can also be achieved through targeted therapies that are based on disruption of the DDR. Such therapies are especially relevant for targeting tumor cells deficient in specific functions of the DNA-damage signaling network.17,18 Therefore, attaining an improved understanding of the increased radiosensitivity observed in HPV-positive oropharyngeal tumors could contribute to the development of novel targeted therapies for radioresistant HNSCC. In HPV-positive oropharyngeal SCC, increased radiosensitivity is related to the HPV-associated perturbations of the cell cycle, activation of the immune system and alterations in DNA-damage repair.14,19, 20, 21 In contrast, radioresistance is associated with multiple cellular adjustments conferring traits such as enhanced DNA repair, altered cell cycle progression, and more efficient ROS scavenging.22,23 Isogenic cell lines with altered radiosensitivity can serve as good experimental and predictive models, as survival differences can be directly attributed to specific modifications in intrinsic cellular features.
We previously established a radioresistant HNSCC cell line from parental FaDu cells via repeated exposure to radiation.23 Additionally, a radiosensitive HPV-positive 2A3 cell line was established from the parental FaDu cells by stable transfection with HPV-16 oncogenes E6 and E7.24 The aim of this study was to exploit these isogenic parental, radioresistant and radiosensitive HNSCC cell lines to evaluate the differences among them in the DDR to DNA-damage inducing agents. We investigated the expression of DNA-damage signaling genes in these cell lines in response to DNA damage-inducing agents: radiation, CDDP and BLEO.
Materials and methods
Cell culture
Isogenic human pharyngeal SCC cell lines FaDu, 2A3 and FaDu-RR, differing in radiosensitivity, were cultured in a humidified atmosphere at 37°C and 5% CO2. Cells were grown in advanced Dulbecco’s modified Eagle medium (DMEM; Gibco, Thermo Fisher, MA, USA) supplemented with 5% fetal bovine serum (FBS, Gibco, Thermo Fisher), 10 mM L-glutamine (GlutaMAX, Gibco), penicillin (100 U/mL) (Grünenthal, Germany) and gentamicin (50 mg/mL) (Krka, Slovenia). The cell culture medium for the 2A3 cell line was additionally supplemented with 1 mg/mL G418 disulfate salt solution (Sigma-Aldrich, MO, USA). The parental TP53 mutated cell line FaDu was obtained from ATCC (HTB-43).25 The HPV-positive cell line 2A3 was established by selection of transfected FaDu cells with HPV16 oncogenes E6 and E7 and was obtained as a gift from Prof. Ekaterina Dadachova – the process described in the paper by Harris M, et al.24 The radioresistant cell line FaDu-RR was established in our laboratory from the parental FaDu cells by repeated exposure to radiation as described previously.23
These isogenic cells were authenticated by short tandem repeat profiling using CellCheck 16 – human (IDEXX BioAnalytics, Germany) for the FaDu and FaDu-RR cells and CellCheck 9 – human (IDEXX BioAnalytics) for the 2A3 cells. The genetic profile of the cell lines used in this study was identical to their publicly available genetic profile.
Study design
To evaluate the expression of DNA-damage signaling genes in isogenic FaDu (HPV-negative) and 2A3 (HPV-positive) cells after exposure to radiation or a combination of radiation and a low radiosensitizing dose of CDDP, cells were first exposed to CDDP (0.1 μg/mL, Cisplatin Kabi; Fresenius Kabi AG, Germany) for 4 h. Then, CDDP was aspirated, and fresh media was added to the cells prior to irradiating the cells at 5 Gy as described previously.23 The established groups were designated control (untreated cells), IR (irradiated cells), CDDP (cisplatin-treated cells) and IR+CDDP (cells treated with irradiation and cisplatin).
Based on the observed CDDP resistance in (radioresistant) FaDu-RR cells,23 the expression of DNA-damage signaling genes in isogenic (radioresistant) FaDu-RR and (parental) FaDu cells exposed to either CDDP (1 μg/mL) or BLEO (5 μM) were evaluated. CDDP or BLEO was added to cell culture media for 2 h. Then, the cell culture media containing the drugs were aspirated from the cell cultures, and fresh media was added to the cells, which were then incubated for 5 h. Final concentrations of CDDP (Cisplatin Kabi) and BLEO (Heinrich Marck Nachf GmbH, Germany) were prepared in phosphate-buffered saline. The established groups were designated control (untreated cells), CDDP (cisplatin-treated cells) and BLEO (bleomycin-treated cells). The selection of CDDP and BLEO concentrations was based on our previous studies.26,27
DNA-damage signaling
The Human DNA Damage Signaling Pathway RT2 ProfilerTM PCR Array (PAHS-029Z, Qiagen, Germany), which allows profiling of the expression of 84 DNA-damage signaling genes, was used to study the DDR in response to IR, CDDP or BLEO in isogenic cell lines with different radiosensitivities. Total RNA was isolated from the cells 5 h after the last treatment by using the RNeasy Plus Mini Kit (Qiagen) according to the manufacturer’s instructions. RNA concentration and sample purity (A260/280) were determined spectrophotometrically on an Epoch Microplate Spectrophotomer (BioTek Instruments, USA). cDNA was synthesized from 2 μg total RNA using the RT2 First Strand Kit (Qiagen) according to the manufacturer’s instructions. Reverse transcription-quantitative PCR was carried out using RT2 qPCR SYBR Green ROX Mastermix (Qiagen) and cycling on a QuantStudio 3 Real-time PCR System (Applied Biosystems, USA) as described previously.23
Data analysis and statistics
GeneGlobe Data Analysis Center (Qiagen) was used to identify reference genes with the most stable gene expression under specific experimental conditions. For the analysis of FaDu cells exposed to IR and CDDP, the expression data were normalized to the gene expression of ACTB, B2M, GAPDH, HPRT1 and RPLP0, and for the analysis of 2A3 cells exposed to IR and CDDP, they were normalized to the gene expression of B2M, GAPDH, HPRT1 and RPLP0. For analysis of FaDu cells exposed to CDDP and BLEO, the data were normalized to the gene expression of ACTB, GAPDH and RPLP0, and for the analysis of FaDu-RR cells exposed to CDDP and BLEO, they were normalized to the gene expression of B2M and RPLP0. The geometric mean of the selected genes was used for accurate averaging of the reference genes.28 The ΔΔCT method was used to calculate the fold change in gene expression.29 To identify significantly differentially expressed genes, thresholds of >1.5 (upregulated) or <0.67 (downregulated) fold-change in gene expression and p value less than 0.05 were set. Clustergrams were created in GeneGlobe Data Analysis Center by nonsupervised hierarchical clustering of the entire dataset to generate a heat map with dendrograms indicating coregulated genes within individual samples or across treatment groups based on the magnitude of gene expression and average linkage. The magnitude of gene expression was determined by calculating the 2-ΔCT for each individual gene and normalizing to the average 2-ΔCT of all genes across all samples. Average linkage is a measure of the distance between clusters and was calculated using the average of the distances in the distance/ dissimilarity matrix between all pairs of genes in the two clusters.
GraphPad Prism 8.1.2 (GraphPad Software, Inc., CA, USA) was used for data visualization and statistical analysis. The data were tested for normal distributions using the D’Agostino and Pearson test. Differences in the overall gene expression data were identified by the nonparametric Kruskall-Wallis test followed by Dunn’s multiple comparison test. Differences in the expression of specific genes were identified by Student’s t-test (two-tailed test assuming equal variances between groups), with the replicate 2–ΔΔCT values for each gene in each treatment group compared with those in the control cells from 3 independent experiments. Differences were considered significant at p value less than 0.05. Unless stated otherwise, data are shown as the mean and standard error of the mean (SEM).
Results
DNA-damage signaling in HPV-negative FaDu cells versus HPV-positive 2A3 cell
In the FaDu cells, i.e., the parental, HPV-negative cells, an overall increase in the expression of DNA-damage signaling genes was observed in response to the studied treatments (Figure 1). The most significant changes from control levels were observed in irradiated cells, in which overall gene expression was also significantly increased compared with that of cells exposed to CDDP either alone or in combination with radiation.
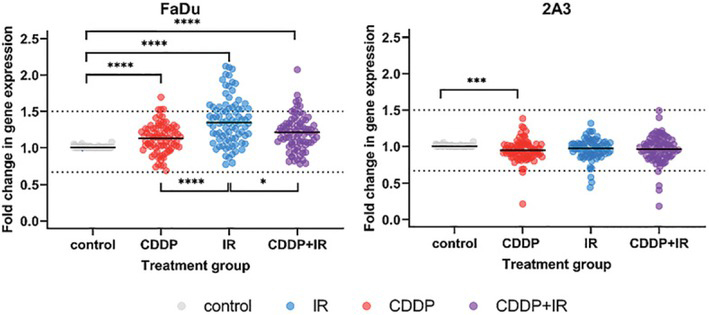
Overall expression of DNA-damage signaling genes in (parental, HPV-negative) FaDu cells and (HPV-positive) 2A3 cells after exposure to cisplatin (CDDP), radiation (IR) or their combination (CDDP+IR). Symbols are mean gene expression for each of the 84 DNA-damage signaling genes included on the Human DNA Damage Signaling Pathway RT2 ProfilerTM PCR Array from 3 independent experiments. The lines indicate the median gene expression of all tested genes.
* p < 0.05; *** p < 0.001; **** p < 0.0001
In the 2A3 cells, i.e., the radiosensitive, HPV-positive cells, the overall expression of DNA-damage signaling genes was less affected by treatment than it was in FaDu cells. A nonsignificant decrease in the expression of DNA-damage signaling genes was observed in comparison to untreated cells (control), with a significant difference in gene expression observed only between control cells and cells exposed solely to CDDP.
Clustergram analysis revealed 9 distinct clusters in (parental) FaDu cells and 10 distinct clusters in (HPV-positive) 2A3 cells at ¼ dendrogram branch height for cells exposed to CDDP, radiation or their combination (Figure 2). The FaDu cells exposed to CDDP either alone or in combination with radiation showed the highest degree of similarity in the expression of DNA-damage signaling genes. Gene expression in these FaDu cells exposed to CDDP, either alone or in combination with radiation, was more similar to that of control cells than to that of irradiated FaDu cells. Similarly, (HPV-positive) 2A3 cells exposed to CDDP either alone or in combination with radiation showed the highest degree of similarity, whereas gene expression in irradiated 2A3 cells was more similar to that of control cells than to that of CDDP-treated cells.
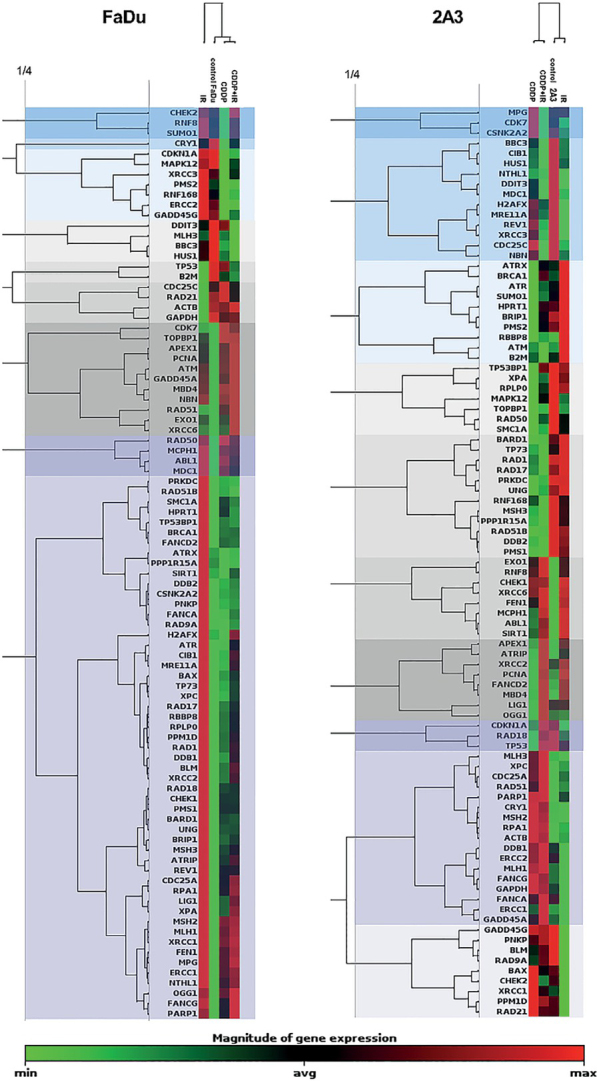
2-D clustergram analysis of the expression of DNA-damage signaling genes in (parental, HPV-negative) FaDu cells and (HPV-positive) 2A3 cells after exposure to cisplatin (CDDP), radiation (IR) or their combination (CDDP+IR). Clusters observed at ¼ dendrogram branch height are shown as blocks of different color.
In (parental, HPV-negative) FaDu cells, 4 genes (NBN, XRCC1, FEN1, and GADD45A) were significantly upregulated after exposure to CDDP, 16 genes (ATR, ATRIP, BARD1, BLM, BRCA1, FANCG, MDC1, MSH3, PMS1, PRKDC, RAD18, RBBP8, REV1, SMC1A, TP73, and UNG) were significantly upregulated after IR, and 6 genes (NBN, XRCC1, FEN1, BLM, EXO1, and GADD45A) were significantly upregulated after exposure to the combination of CDDP and radiation. The seven genes (ATR, ATRIP, BARD1, BRCA1, MDC1, RBBP8 and SMC1A) that were significantly upregulated in response to IR are mainly involved in ATM/ATR signaling (Figure 3).
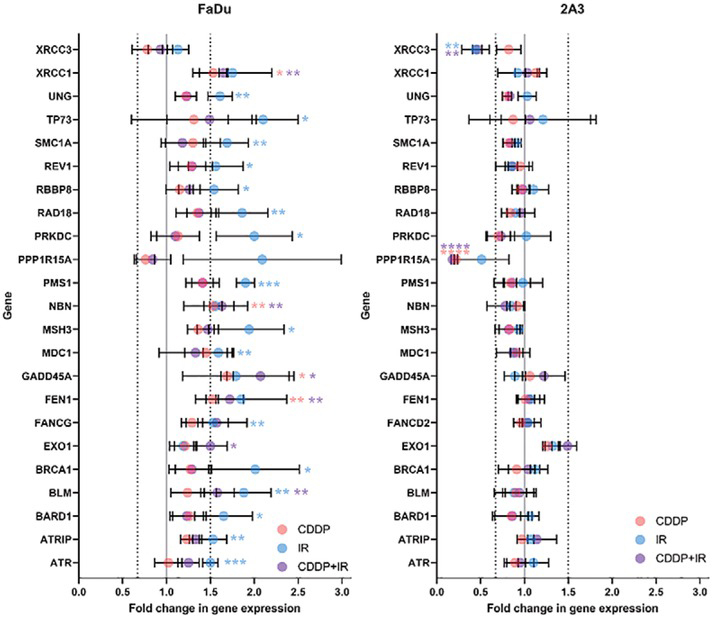
Deregulated expression of DNA-damage signaling genes in (parental, HPV-negative) FaDu cells and (HPV-positive) 2A3 cells after exposure to cisplatin (CDDP), radiation (IR) or their combination (CDDP+IR). Only significantly deregulated genes are shown. Symbols are the mean and SEM from three independent experiments.
* p < 0.05; ** p < 0.01; *** p < 0.001
In (HPV-positive) 2A3 cells, no upregulation of DNA-damage signaling genes and no significant changes in the expression of ATM/ATR signaling genes were observed in response to CDDP, IR or CDDP+IR treatment. One gene (PPP1R15A) was significantly downregulated after exposure to CDDP, 1 gene (XRCC3) was significantly down-regulated after IR, and 2 genes (PPP1R15A and XRCC3) were significantly downregulated after CDDP+IR (Figure 3).
The expression of genes involved in various repair pathways was significantly upregulated in response to CDDP, IR or CDDP+IR in (parental, HPV-negative) FaDu cells but not in (HPV-positive) 2A3 cells. The major DDR gene involved in the initiation of the DDR and HR pathway repair, NBN, was upregulated after exposure to CDDP, both alone and in combination with radiation. Furthermore, 4 genes involved in the initial steps of the DNA-damage response and the HR repair pathway (BLM, BRCA1, MDC1 and PRKDC) were upregulated in response to IR; among them, BLM was also upregulated in response to CDDP+IR treatment. The expression of nucleotide excision repair (NER) genes was not significantly affected in either (parental, HPV-negative) FaDu cells or (HPV-positive) 2A3 cells in response to CDDP, IR or CDDP+IR treatment. The expression of base excision repair (BER) genes was not significantly affected in (HPV-positive) 2A3 cells in response to any of treatment. In FaDu cells, expression of the BER gene XRCC1 and the microhomology-mediated end joining (MMEJ) gene FEN1 was significantly upregulated in response to both CDDP and CDDP+IR, whereas nonsignificant upregulation of these two genes was observed after IR alone. Gene expression of MSH3, PMS1 and TP73, involved in mismatch repair (MMR), was significantly upregulated in (parental, HPV-negative) FaDu cells after IR. In (HPV-positive) 2A3 cells, upregulation of EXO1 was observed after exposure to CDDP and IR. In addition, the expression of other repair genes was significantly affected by treatment in FaDu cells. Specifically, 8 genes (ATR, ATRIP, BARD1, FANCG, RAD18, RBBP8, REV1, and SMC1A) were significantly upregulated after IR, whereas GADD45A was significantly upregulated after exposure to CDDP or CDDP+IR. In (HPV-positive) 2A3 cells, an important gene for HR, XRCC3, was significantly downregulated after IR or CDDP+IR.
The genes ATR, ATRIP, MDC1 and TP73, which are involved in cell cycle regulation, were significantly upregulated in (parental, HPV-negative) FaDu cells after IR. Additionally, the expression of PPP1R15A in these cells was upregulated 2.1-fold after IR. In contrast, in (HPV-positive) 2A3 cells, PPP1R15A was significantly downregulated after exposure to CDDP or CDDP+IR and was down-regulated 2-fold after IR.
The expression of 4 genes (BARD1, BRCA1, PRKDC and TP73), involved in apoptosis, was significantly upregulated after IR in (parental, HPV-negative) FaDu cells. Additionally, the expression of PPP1R15A in these cells was upregulated 2.1-fold (p=0.17) after IR. On the other hand, in (HPV-positive) 2A3 cells, PPP1R15A was significantly downregulated after exposure to CDDP either alone or in combination with radiation. Furthermore, the expression of PPP1R15A was downregulated 2-fold (p=0.18) after IR.
DNA-damage signaling in (parental) FaDu cells versus (radioresistant) FaDu-RR cells
Gene expression in (parental, HPV-negative) FaDu cells exposed to either CDDP or BLEO did not differ significantly from that in untreated (control) cells (Figure 4). After exposure to CDDP, cells of the radioresistant line, FaDu-RR, showed a significantly different pattern of gene expression from control or BLEO-treated cells.
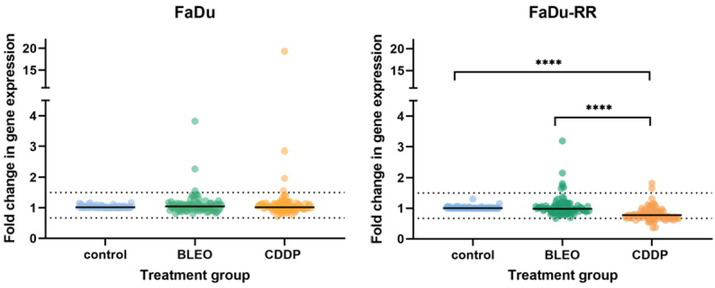
Overall gene expression in (parental, HPV-negative) FaDu cells and (radioresistant) FaDu-RR cells in response to cisplatin (CDDP) and bleomycin (BLEO). Symbols are mean gene expression for each of the 84 DNA-damage signaling genes included on the Human DNA Damage Signaling Pathway RT2 ProfilerTM PCR Array from 3 independent experiments. The lines indicate the median gene expression of all tested genes.
* p < 0.05; *** p < 0.001; **** p < 0.0001
Clustergram analysis revealed 6 distinct clusters in (parental) FaDu cells and 5 distinct clusters in (radioresistant) FaDu-RR cells at ¼ dendrogram branch height in cells exposed to CDDP or BLEO (Figure 5). CDDP- and BLEO-exposed (parental) FaDu cells were more similar to each other in the expression of DNA-damage signaling genes than they were to control FaDu cells. The expression of DNA-damage signaling genes in BLEO-exposed FaDu-RR cells was more similar to that in control cells than to that in CDDP-exposed cells.
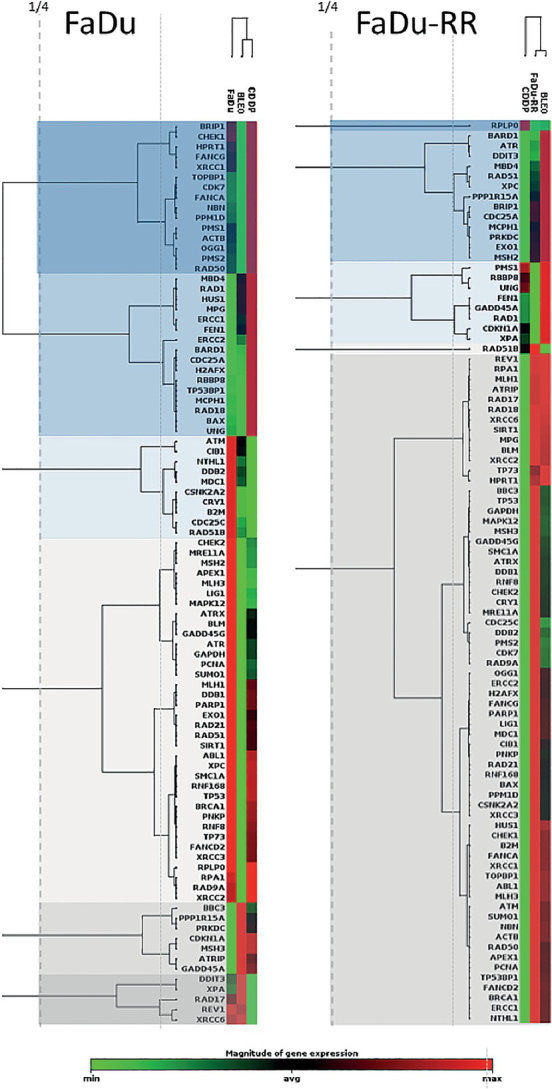
2-D clustergram analysis of the expression of DNA-damage signaling genes in (parental) FaDu cells and (radioresistant) FaDu-RR cells after exposure to cisplatin (CDDP) or bleomycin (BLEO). Clusters observed at ¼ dendrogram branch height are shown as blocks of different color.
In total, in (parental) FaDu cells, 2 genes (CDKN1A, GADD45A) were significantly upregulated after exposure to CDDP or BLEO. In (radioresistant) FaDu-RR cells, 1 gene (CDC25C) was significantly downregulated after exposure to CDDP, and 1 gene (XPA) was significantly upregulated after exposure to BLEO.
In (parental) FaDu cells, none of the genes involved in the ATM/ATR signaling pathway were significantly affected by exposure to CDDP or BLEO. Furthermore, no significant changes in the expression of ATM/ATR signaling genes were observed in response to CDDP or BLEO in (radioresistant) FaDu-RR cells. No genes involved in DSB and BER were significantly deregulated in (parental) FaDu or (radioresistant) FaDu-RR cells after exposure to CDDP or BLEO. NER gene expression in (parental) FaDu cells was unaffected by exposure to BLEO or CDDP, whereas in (radioresistant) FaDu-RR cells, XPA was significantly upregulated in response to BLEO. MMR genes were not significantly deregulated in (radioresistant) FaDu-RR cells after exposure to CDDP or BLEO. The expression of other repair genes was deregulated in (parental) FaDu cells but not in (radioresistant) FaDu-RR cells. GADD45A expression was significantly upregulated after exposure to CDDP or BLEO.
In response to CDDP or BLEO, CDKN1A was significantly upregulated in (parental) FaDu cells, and CDC25C was significantly downregulated after exposure to CDDP in (radioresistant) FaDu-RR cells.
In response to CDDP or BLEO, CDKN1A, involved in apoptosis, was significantly upregulated while TP73 was significantly downregulated in (parental) FaDu cells. In (radioresistant) FaDu-RR cells, gene expression of CDKN1A was nonsignificantly upregulated after exposure to CDDP or BLEO (Figure 6).
Discussion
Genomic instability is one of the hallmarks of cancer and is associated with the accumulation of DNA damage.30 Targeting the DDR by tailored cancer therapy offers a possibility to broaden the therapeutic window.17 After exposure to DNA-damaging agents, the DDR plays a central role in the sensitivity of cells and tissues to these toxic agents and, importantly, it affects the therapeutic outcome. Efficient DDR after therapy with DNA-damaging agents promotes resistance, whereas DDR defects can lead to increased cell death and sensitivity to treatment.31 In radiotherapy of HNSCC both phenomena, increased radioresistance and increased radiosensitivity, can be observed in specific different entities. The radioresistant HNSCC typically arises in previously irradiated areas, and any additional treatment is associated with a high risk of normal tissue toxicity, impaired quality of life and poor outcome.9,10 Compared to tobacco- and alcohol-induced HPV-negative tumors, HPV-positive oropharyngeal SCCs display an increased sensitivity to radio- and chemotherapy and are associated with reduced risk of locoregional recurrence and increased survival probability.32, 33, 34, 35, 36
For the purposes of the present study, we used the commercially available RT2 Profiler PCR Array, which has been designed to evaluate the expression of 84 genes involved in DNA-damage signaling or ATM/ATR signaling, DNA repair, cell cycle and apoptosis. These arrays are designed to enable a quick and reliable gene expression analysis of relevant genes from specific pathway, and represent an important first step in screening for differentially expressed genes under specific conditions as an alternative to more expensive and complex microarray analysis. These assays are accurate and easy to use.
The expression of the studied genes varied with HPV status. DNA damage signaling and repair gene expression in HPV-negative (FaDU) and HPV-positive (2A3) cells in response to CDDP, IR and CDDP+IR was different according to the HPV status. In the parental HPV-negative (FaDu) cells, we observed significantly increased expression of DNA-damage signaling and HR repair pathway genes after CDDP+IR treatment. The opposite trend was observed in HPV-positive (2A3) cells, which exhibited patterns of DNA-damage signaling and repair gene expression similar to those of nonirradiated control 2A3 cells.
Increased expression of ATM/ATR signaling genes was observed in (parental, HPV-negative) FaDu cells in response to IR but not in response to CDDP either alone or in combination with IR. On the other hand, in (HPV-positive) 2A3 cells, no change in ATM/ATR signaling gene expression was detected after cisplatin, IR or combination CDDP+IR treatment. ATM is primarily activated by DNA DSBs, whereas ATR is activated in response to various DNA lesions that interfere with DNA replication, such as stalled replication forks, but also DSB.1,37 ATM is therefore required for the early response to IR-induced DSB and cells lacking ATM are extremely sensitive to IR.38 Although the upregulation of ATR and ATRIP can be expected due to their involvement in the HR, it could also indicate the presence of ssDNA, since the protein complex ATR-ATRIP is recruited to sites containing single-strand DNA regions.38,39 Increased sensitivity to CDDP and IR upon inhibition of ATR has been previously shown in several studies on head and neck squamous cell carcinoma cells in vitro.40, 41, 42
Previously, we observed cell cycle delay in S and G2/M phase in FaDu and 2A3 cells 5 h after IR.23 Such delay could be due to HR repair of DSBs in FaDu cells, as indicated by the upregulation of some specific DNA-damage signaling and repair genes (BRCA1, BARD1, RBBP8, and SMC1A) observed in this study. The NBN gene for NBS1 protein is a major factor involved in the earliest stages of DSB detection as part of the MRE11-RAD50-NBS1 protein and as a DNA repair protein of HR pathway.43 NBN expression did not differ among untreated (control) isogenic FaDu, FaDu-RR and 2A3 cells; however, a difference in NBN expression was found in response to IR, with upregulation observed in (radioresistant) FaDu-RR cells and downregulation observed in the radiosensitive (HPV-positive) 2A3 cells.23 Similarly, Prati et al. observed downregulation of NBN and XRCC2 (the latter being a gene for HR paralog protein, similar to XRCC3, discussed below) in HPV-positive cervical cancer cells in comparison to HPV-negative cells.44 In the paper by Girard PM, et al., cellular radiosensitivity in cells lacking NBS1 protein has been established due to repair defect.45 In (HPV-positive) 2A3 cells, gene XRCC3 was significantly downregulated after IR, and significantly downregulated after CDDP+IR. XRCC3 is a major HR protein and the levels of the protein strongly correlate with response to radiation. For example, Cheng J, et al., observed a substantial increase in radiosensitivity of esophageal SCC with knock-down of XRCC3.46 BARD1 and BRCA1 are extremely important HR regulators, and their deficiency results in HR defect.1,47 RBBP8 is an important factor in the NHEJ-to-HR switch.48,49 SMC1A is one of the four subunits of cohesin, a protein complex promoting DNA repair by HR and the recruitment of proteins involved in the activation of S and G2/M-checkpoints.50 The present data imply that these genes play important roles in HR repair in HPV-negative FaDu cells. This implication should be confirmed at the protein level by Western blotting; the lack of confirmation here is one of the limitations of the present study. Since no alteration in gene expression in (HPV-positive) 2A3 cells was observed at 5 h after IR, it is possible that the DSBs observed in our previous study at 5 h after IR remained unrepaired,23 due to either damage complexity or inactive/insufficient HR repair, and that such breaks are responsible for the increased radiosensitivity of (HPV-positive) 2A3 cells. MDC1 is required for full size formation of gH2AX foci at DSB sites.1 The upregulation of MDC1 in (HPV-negative) FaDu cells but not (HPV-positive) 2A3 cells could thus indicate a limited formation of gH2AX foci in 2A3 cells, which would impair DSB repair. More efficient DSB repair was observed in FaDu cells than 2A3 cells after IR in the slow phase of DSB repair, which corresponds to the HR repair of DSBs.23
In addition to DSBs, other types of IR-associated DNA damage are repaired by BER.17 The anticancer effect of IR can be potentiated by CDDP.14,51 CDDP-induced damage to the nucleotides causes distortions in DNA structure and can be repaired by NER.17 In the present study, the expression of the NER and BER genes was not affected in HPV-positive 2A3 cells. In (parental, HPV-negative) FaDu cells, the BER gene XRCC1 and the MMEJ gene FEN1 were upregulated in response to CDDP. The lack of change in BER gene expression in the HPV-positive cells indicates the possible presence of unrepaired single-strand breaks, abasic sites and modified bases, including damaged bases by ROS. In HPV-positive cells compared with HPV-negative cells, more oxidative damage is expected due to E6-mediated oxidative stress.52
Alterations in DNA-damage signaling are associated with the onset of radioresistance. In addition to radioresistance, CDDP cross-resistance has been observed in some radioresistant cell lines.23,53, 54, 55, 56, 57, CDDP resistance is multifactorial and can be classified into the following types: pretarget resistance, affecting the intracellular accumulation of CDDP; on-target resistance, directly influencing CDDP-DNA adducts; posttarget resistance, affecting DNA-damage signaling pathways in response to CDDP-induced DNA damage; and off-target resistance, affecting signaling pathways not directly linked to CDDP-induced damage.4,5 It is not clear how much each of these resistance mechanisms contributes to overall CDDP resistance. However, the application of CDDP in assumed radiosensitive tumors should be carefully considered, and alternative approaches might have better therapeutic effect.
Posttarget CDDP resistance includes altered DNA-damage signaling pathways in response to CDDP-induced DNA damage. Interestingly, we have observed significantly altered expression of DNA-damage signaling genes after exposure to CDDP in radioresistant and CDDP-resistant FaDu-RR cells but not in (parental, HPV-negative) FaDu cells. Altered expression of DNA-damage signaling genes was confirmed by clustergram analysis, which revealed that in (radioresistant) FaDu-RR cells, gene expression after CDDP exposure was dissimilar to that after exposure to BLEO. Overall, a trend of downregulated DNA-damage signaling genes was observed in (radioresistant) FaDu-RR cells in comparison to control (untreated) cells.
CDKN1A and GADD45A were upregulated in response to CDDP and BLEO in (parental) FaDu cells, whereas their expression was increased to a lesser degree in (radioresistant) FaDu-RR cells. Transcriptional upregulation of these genes can mediate cell cycle arrest.58 GADD45A plays roles in cell cycle regulation, DNA repair and apoptosis and is associated with increased transcriptional activity.59, 60, 61 GADD45 proteins act as stress sensors and tumor suppressor proteins. After cellular exposure to toxic agents – e.g. IR or alkylating agent, the members of this protein family are induced. This can cause cell cycle arrest and/or DNA repair induction. The role of GADD45 proteins in the DNA repair machinery is still not very clear, but important proteins of cell cycle arrest and DNA repair, such as p53 and BRCA1 (a protein of HR pathway) are known to regulate GADD45 expression by increasing transcription of the GADD45 gene expression.62 Its role in CDDP resistance is unclear, as silencing of GADD45A can either reduce or increase CDDP sensitivity.63,64 Interestingly, after exposure to IR or alkylating agents, cells lacking p53 protein exhibits reduced levels of GADD45 activity. Also, following DNA damage GADD45A stabilizes p53 protein, an important step towards increased p53 activity. It has been reported that GADD45 null mice display increased sensitivity to radiation carcinogenesis and genomic instability.62,65
CDKN1A encodes cyclin-dependent kinase inhibitor p21, which acts as a principal mediator of cell cycle arrest in response to DNA damage and as a promoter of apoptosis and transcriptional activity.66 It is directly downstream of p53 protein.67
Upregulation of XPA was observed after exposure to BLEO in (radioresistant) FaDu-RR cells. After exposure to CDDP, XPA gene expression was increased, but the increase did not reach significance. In (parental) FaDu cells, XPA was not significantly increased. Upregulation of XPA has also been observed in radioresistant glioblastoma cells.68 XPA upregulation could indicate active DNA repair through NER, since XPA plays a central role in this repair pathway, via which CDDP-DNA adducts are repaired. This speculation is supported by the increased expression of XPC observed in FaDu-RR cells. Both XPC and XPA are part of protein complexes that participate in the recognition of DNA interstrand crosslinks.69 XPA is also involved in CDDP resistance; by targeting the NER pathway, specifically, its central player XPA, sensitization to CDDP can be achieved.70,71
Most of the up or down regulated genes determined in our study are connected to p53 protein, which is inactivated in about 80% of HNSCC,72 thus representing a major player in the HNSCC carcinogenesis. Increased expression of ATR, which was observed in FaDu cells in response to IR, could lead to increased phosphorylation mediated by ATR kinase and thus stabilization of p53 protein, leading to quiescence or senescence.73 NBN expression, for example was down-regulated in 2A3 cells, and up-regulated in FaDu-RR cells in response to IR. NBN modulates the DNA damage signal sensing also by recruiting PI3/PI4-kinase family members ATM and ATR and further influencing the TP53 expression.74 On the other hand, p53 regulates the transcription of cell cycle genes, such as GADD45A gene, which was up-regulated in response to treatment with chemotherapeutics and IR in FaDu and FaDu-RR cell, but less in 2A3 cells, again in line with phenotypic features of radiosensitivity of these isogenic cell lines. Furthermore, upregulation of XPA, which is involved in nucleotide excision repair, was observed after treatment of FaDu-RR cells with chemotherapeutics, that could also be the result of p53 stabilization.
To conclude, the use of predesigned panels of DNA-damage signaling and repair genes proved to be a convenient approach for quickly identifying possible therapeutic targets in isogenic HNSCC cells lines with different degrees of radiosensitivity. Based in the described results, we identified several interesting targets, such as NBN, XRCC3, ATR, GADD45A and XPA, that could be exploited for the potentiation of the antitumor effects of IR and/or CDDP in resistant (HPV-negative) tumor cells. Among these genes, NBN and XRCC3 are of particular interest due to their roles in the initiation of DSB recognition and response and in HR activation. One of the major limitations of the presented study is the lack of the evidence at the protein level. Using western blotting technique would allow us to confirm and validate the results at the protein expression level. Also, another approach to validate the feasibility of these potential targets should be the knock-down of genes of interest.
Acknowledgments
The language edition was performed by American Journal Experts. The authors also acknowledge the financial support from the state budget by the Slovenian Research Agency (program No. P3-0307).
References
Articles from Radiology and Oncology are provided here courtesy of De Gruyter
Full text links
Read article at publisher's site: https://doi.org/10.2478/raon-2022-0014
Read article for free, from open access legal sources, via Unpaywall:
https://sciendo.com/pdf/10.2478/raon-2022-0014
Citations & impact
Impact metrics
Citations of article over time
Alternative metrics

Discover the attention surrounding your research
https://www.altmetric.com/details/126149139
Article citations
HPV-positive murine oral squamous cell carcinoma: development and characterization of a new mouse tumor model for immunological studies.
J Transl Med, 21(1):376, 10 Jun 2023
Cited by: 1 article | PMID: 37296466 | PMCID: PMC10257320
Tumor Radiosensitization by Gene Electrotransfer-Mediated Double Targeting of Tumor Vasculature.
Int J Mol Sci, 24(3):2755, 01 Feb 2023
Cited by: 1 article | PMID: 36769077 | PMCID: PMC9917180
Association between XRCC3 rs861539 Polymorphism and the Risk of Ovarian Cancer: Meta-Analysis and Trial Sequential Analysis.
Biomed Res Int, 2022:3915402, 08 Aug 2022
Cited by: 2 articles | PMID: 35978646 | PMCID: PMC9377891
Review Free full text in Europe PMC
Similar Articles
To arrive at the top five similar articles we use a word-weighted algorithm to compare words from the Title and Abstract of each citation.
Mechanisms of different response to ionizing irradiation in isogenic head and neck cancer cell lines.
Radiat Oncol, 14(1):214, 27 Nov 2019
Cited by: 13 articles | PMID: 31775835 | PMCID: PMC6882348
Pulsed low dose-rate irradiation response in isogenic HNSCC cell lines with different radiosensitivity.
Radiol Oncol, 54(2):168-179, 27 Mar 2020
Cited by: 6 articles | PMID: 32229678 | PMCID: PMC7276640
Transcriptomic analyses of the radiation response in head and neck squamous cell carcinoma subclones with different radiation sensitivity: time-course gene expression profiles and gene association networks.
Radiat Oncol, 11:94, 26 Jul 2016
Cited by: 26 articles | PMID: 27455841 | PMCID: PMC4960706
The radiobiology of HPV-positive and HPV-negative head and neck squamous cell carcinoma.
Expert Rev Mol Med, 22:e3, 02 Jul 2020
Cited by: 29 articles | PMID: 32611474 | PMCID: PMC7754878
Review Free full text in Europe PMC