Abstract
Free full text

Kidney Angiotensin in Cardiovascular Disease: Formation and Drug Targeting
Abstract
The concept of local formation of angiotensin II in the kidney has changed over the last 10–15 years. Local synthesis of angiotensinogen in the proximal tubule has been proposed, combined with prorenin synthesis in the collecting duct. Binding of prorenin via the so-called (pro)renin receptor has been introduced, as well as megalin-mediated uptake of filtered plasma-derived renin-angiotensin system (RAS) components. Moreover, angiotensin metabolites other than angiotensin II [notably angiotensin-(1-7)] exist, and angiotensins exert their effects via three different receptors, of which angiotensin II type 2 and Mas receptors are considered renoprotective, possibly in a sex-specific manner, whereas angiotensin II type 1 (AT1) receptors are believed to be deleterious. Additionally, internalized angiotensin II may stimulate intracellular receptors. Angiotensin-converting enzyme 2 (ACE2) not only generates angiotensin-(1-7) but also acts as coronavirus receptor. Multiple, if not all, cardiovascular diseases involve the kidney RAS, with renal AT1 receptors often being claimed to exert a crucial role. Urinary RAS component levels, depending on filtration, reabsorption, and local release, are believed to reflect renal RAS activity. Finally, both existing drugs (RAS inhibitors, cyclooxygenase inhibitors) and novel drugs (angiotensin receptor/neprilysin inhibitors, sodium-glucose cotransporter-2 inhibitors, soluble ACE2) affect renal angiotensin formation, thereby displaying cardiovascular efficacy. Particular in the case of the latter three, an important question is to what degree they induce renoprotection (e.g., in a renal RAS-dependent manner). This review provides a unifying view, explaining not only how kidney angiotensin formation occurs and how it is affected by drugs but also why drugs are renoprotective when altering the renal RAS.
Significance Statement
Angiotensin formation in the kidney is widely accepted but little understood, and multiple, often contrasting concepts have been put forward over the last two decades. This paper offers a unifying view, simultaneously explaining how existing and novel drugs exert renoprotection by interfering with kidney angiotensin formation.
I. Introduction
Angiotensin (Ang) II exerts multiple effects in the kidney (Kobori et al., 2007b), and it is now widely accepted that this involves locally synthesized rather than circulating Ang II. The beneficial effects of renin-angiotensin system (RAS) blockers in the kidney, often occurring independently of their blood pressure–lowering effects, support this view. Yet, how exactly this independent angiotensin generation occurs remains incompletely understood. For many years, it was thought to depend on angiotensinogen (AGT) synthesis in the kidney, combined with local renin synthesis in the collecting duct (CD). The discovery of a receptor for prorenin, the so-called (pro)renin receptor [(P)RR] (Nguyen et al., 2002), caused further excitement, as this might explain why the CD predominantly generated prorenin (i.e., renin’s inactive precursor). By binding to this receptor, prorenin would be able to display activity, and it even seemed to act as an agonist of this receptor. Hence, the (P)RR was welcomed as a new RAS member. Other members received wide attention as well, in particular the previously less studied Ang II type 2 (AT2) and Mas receptor. The latter recognizes Ang-(1-7). Remarkably, these two receptors are now believed to oppose the classic, deleterious effects of Ang II in the kidney, mediated via its type 1 (AT1) receptor. To our knowledge, this has not yet led to novel drugs for cardiovascular or kidney disease.
The presence of Ang-(1-7) in the kidney requires an enzyme capable of generating this metabolite in sufficient quantities. Here angiotensin-converting enzyme 2 (ACE2) comes into play. Given its additional role as coronavirus receptor, the relationship between the RAS, its inhibitors, and coronavirus disease 2019 (COVID-19) is currently hotly debated (Danser et al., 2020) and novel treatment options like soluble ACE2 are likely to emerge. Angiotensin receptor/neprilysin inhibition (ARNI) and sodium-glucose cotransporter-2 (SGLT2) inhibition, originally introduced for the treatment of heart failure and diabetes respectively, turned out to be renoprotective as well, possibly by affecting the renal RAS. This is also true for another group of drugs, the cyclooxygenase (COX) inhibitors, and novel drugs capable of suppressing AGT generation. The latter, by acting in a liver-specific manner, have helped to address the origin of tissue AGT and have also altered our view on urinary AGT as a biomarker (Roksnoer et al., 2016a).
This review provides an up-to-date review of the renal RAS, focusing primarily on how it allows/facilitates local angiotensin generation in health and disease. We start by evaluating the various concepts of renin synthesis in the kidney at its classic location in the juxtaglomerular (JG) cells versus the CD, simultaneously considering the role of the (P)RR. We then address the origin of renal AGT and discuss the various enzymes involved in kidney angiotensin generation and metabolism, including their local expression, as well as the three major angiotensin receptors. Next, the renal RAS in hypertension, kidney disease, and metabolic disease is discussed, critically addressing the role of urinary AGT as a biomarker reflecting renal RAS activity. Finally, the review summarizes how novel and existing drugs (RAS blockers, ACE2 activators, ARNI, COX inhibitors, and SGLT2 inhibitors) affect the renal RAS, paying particular attention to how kidney angiotensins should be measured and what their in vivo levels truly are. We do not discuss β-adrenergic antagonists, calcium antagonists, and diuretics since their effects on the RAS have already been extensively described (Kobori et al., 2007b).
II. Intrarenal Angiotensin Generation: Location, Enzymes, Substrates, and Receptors
A. Prorenin, Renin, and (Pro)renin Receptor: Juxtaglomerular Cells
Prorenin is the inactive precursor of renin. It contains a 43-amino-acid prosegment covering the active site (Danser and Deinum, 2005). Although both renin and prorenin are synthesized and secreted by JG cells, only renin is capable of cleaving angiotensin (Ang) I from AGT. Yet, the kidneys secrete much more prorenin, and generally the circulating prorenin levels are 10-fold higher than those of renin (Danser et al., 1998). The latter is also related to the fact that other organs, including the ovaries, testes, and adrenals (Krop and Danser, 2008), secrete prorenin as well. Prorenin can be activated in a proteolytic or nonproteolytic manner. Proteolytic activation occurs in renin-synthesizing cells and involves the actual removal of the prosegment by a proconvertase (e.g., kallikrein, trypsin, or cathepsin G). This process is irreversible. Acidic pH, low temperature, and receptor binding are capable of reversibly inducing a conformational change in the prorenin molecule, allowing exposure of the active site (Danser and Deinum, 2005) (Fig. 1).
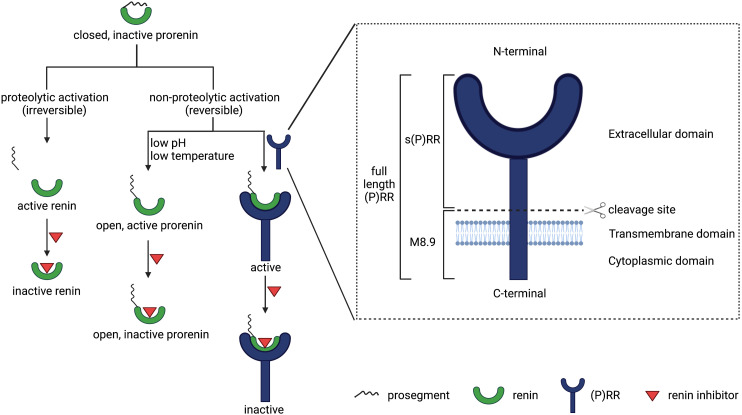
Overview of all (pro)renin forms, prorenin activation, and the (pro)renin receptor in all its roles.
Renin is synthesized primarily by JG cells located in the renal afferent arteriole. Potential additional sources of renin are renal mesangial cells, arteriolar vascular smooth muscle cells (VSMCs), interstitial pericytes, and tubuloepithelial cells (Brunskill et al., 2011; Sequeira-Lopez et al., 2015). The three major mechanisms that control renin synthesis and release into the circulation are β1-adrenergic receptors, macula densa paracrine signaling, and the renal baroreceptor. Indeed, sympathetic stimulation, decreased NaCl concentrations sensed by the macula densa, and a reduced perfusion pressure detected by renal baroreceptors upregulate renin synthesis and release (Gomez and Sequeira-Lopez, 2018). Here, the second messenger cAMP, generated by adenylate cyclase, plays a critical role, as it leads to phosphorylation of the transcription factor CREB (cAMP-responsive element-binding protein). CREB binds to the cAMP-responsive element at the renin locus regulatory region. Access to this region is determined by the histone acetyltransferases CREB-binding protein and p300, which elicit the deposition of acetylation of histones around the cAMP-responsive element, thus resulting in the displacement of nucleosomes and opening of chromatin for CREB and allowing transcription of the renin gene (Sequeira-Lopez and Gomez, 2021). Importantly, Ang II inhibits renin synthesis and release by JG cells via the AT1 receptor, thereby creating a negative feedback loop within the RAS.
Renin-expressing cells emerge in renal and extrarenal tissues during embryonic development (Sequeira López et al., 2004). Renin progenitor cells consisting of JG cells, mesangial cells, VSMCs, and pericytes derive from forkhead box protein D1 (Foxd1)-expressing progenitors within the stromal compartment (Sequeira-Lopez et al., 2015). In fetal mammalian kidneys, renin-producing cells exist along the afferent arterioles of the glomerulus and in the mesangium. In adult mammals, renin-producing cells are confined to the JG area, whereas those in the arterioles and mesangium differentiate into VSMCs and mesangial cells (Sequeira-Lopez and Gomez, 2021). Given the function of the RAS, renin-producing cells are vital for survival and homeostatic maintenance in the body. Further, they also play an essential role in the morphogenesis of the renal arteriolar tree and the regeneration of injured glomerular cells (Gomez and Sequeira-Lopez, 2018; Guessoum et al., 2021; Sequeira-Lopez and Gomez, 2021). Three major factors control the differentiation and maintenance of renin cells. In addition to the aforementioned cAMP/CREB-binding protein/p300 pathway, the Notch/RBP-J pathway and the transcription factor Foxd1 are also crucial for the development of renin cells and kidney vasculature (Lin et al., 2014; Castellanos-Rivera et al., 2015). Ablation of RBP-J in renin-producing cells or Foxd1+ stromal cells caused a reduction in the number of renin-producing cells and abnormal renal vasculature (Castellanos Rivera et al., 2011; Lin et al., 2014; Castellanos-Rivera et al., 2015). Furthermore, renin cells rely on the interaction with adjacent cells to maintain their phenotype. Connexin 40 and β1-integrin play an important role in the communication between renin cells and other cell types (Sequeira-Lopez and Gomez, 2021). Connexin 40 helps to anchor renin cells to JG sites and to control renin synthesis and release. Conditional deletion of Connexin 40 in renin-expressing cells led to mislocalization of JG renin cells and excessive secretion of renin, resulting to malignant hypertension (Wagner et al., 2007). β1-integrin is required for the development and function of renin cells, and its absence caused a marked decrease in the number of renin cells and vascular alterations (Mohamed et al., 2020).
Renin lineage cells retain the plasticity and developmental memory to reexpress renin when faced with homeostatic threats such as hypotension, fluid-electrolyte imbalance, and hypoxia (Sequeira López et al., 2004). Indeed, in response to homeostatic challenges, not only JG cells start to synthesize and secrete more renin, but simultaneously VSMCs along the renal arterioles transform into renin cells by remodeling their chromatin to carry activating epigenetic marks such as H3K27Ac, thereby allowing binding of transcription factors (Guessoum et al., 2021). Super-enhancers unique to renin cells serve as chromatin sensors for the maintenance of renin-expressing cell identity and memory (Sequeira-Lopez and Gomez, 2021). Once homeostasis is restored, the transformed cells return to their VSMC phenotype. However, when homeostasis fails to be restored (e.g., due to chronic stimuli such as RAS blockade, hypotension, and salt depletion), the progressive transformation of arteriolar cells occurs constantly and the renin program is inappropriately overactivated, leading to concentric vascular hypertrophy (Gomez and Sequeira-Lopez, 2018; Guessoum et al., 2021; Sequeira-Lopez and Gomez, 2021). Hence, the deletion of RAS genes or chronic RAS inhibition in both mice and humans causes concentric arterial and arteriolar hypertrophy accompanied by the accumulation of renin cells (Oka et al., 2017; Guessoum et al., 2021; Watanabe et al., 2021). Ablation of renin cells by either conditional β1-integrin deletion or diphtheria toxin prevents this phenomenon (Pentz et al., 2004; Watanabe et al., 2021), indicating that renin cells are responsible for vascular hypertrophy.
The (P)RR, also known as ATP6AP2, is a 350-amino-acid receptor protein, which was cloned by Nguyen et al. (2002). It is composed of a N-terminal extracellular domain, a transmembrane domain, and a C-terminal cytoplasmic domain (Burcklé and Bader, 2006). There are three forms of (P)RR: the 39 kDa full-length (P)RR, the 28 kDa soluble (P)RR [s(P)RR], and the 8.9 kDa M8.9 (Fig. 1). Soluble (P)RR is generated via cleavage of the full-length (P)RR by either furin (Cousin et al., 2009), a disintegrin and metalloproteinase (ADAM)-19 (Yoshikawa et al., 2011), or site 1 protease (Nakagawa et al., 2017). The (P)RR is ubiquitously expressed (Nguyen et al., 2002) and in the kidney occurs in the macula densa, mesangial cells, podocytes, proximal tubule, and CD (Advani et al., 2009; Gonzalez et al., 2011; Gonzalez et al., 2013). Its name is based on the observation that it binds both renin and prorenin and allows prorenin to undergo the conformational change described above, rendering it active. Since prorenin binds with greater affinity (Batenburg et al., 2007), the (P)RR was initially welcomed as the receptor of prorenin that finally explained why we have such high prorenin levels: the inactive prorenin would display activity at tissue sites, allowing angiotensin generation in case of local (P)RR expression. Exciting data were obtained with the putative (P)RR antagonists handle region peptide and PRO20 (Ichihara et al., 2004), which both mimic the parts of the prorenin prosegment that are believed to interact with the (P)RR. Rigorous testing of these antagonists never occurred, and until today no convincing data exist that support that these tools truly represent (P)RR antagonists. In fact, the few studies that did investigate this found no evidence for any antagonistic properties of handle region peptide (Feldt et al., 2008; te Riet et al., 2014). A further complicating factor is that the binding affinity of the (P)RR for both renin and prorenin is many orders of magnitude below its in vivo levels, thus making a meaningful interaction in vivo unlikely (Batenburg et al., 2011), except possibly at sites where prorenin is produced locally. Simultaneously, over the last 10–15 years, multiple alternative RAS-independent functions for the (P)RR as a subunit of vacuolar H+-adenosine triphosphatase (ATPase) have been discovered related to autophagy (Kinouchi et al., 2010), Wnt/β-catenin signaling, embryonic development, and cell differentiation (Cruciat et al., 2010), as well as lipid metabolism and energy homeostasis (Ren et al., 2018). Moreover, (P)RR deletion is lethal (Kinouchi et al., 2010), whereas RAS gene deletion is not. As a consequence, the concept that the (P)RR is the endogenous receptor of prorenin at extrarenal sites is now being reevaluated (Sun et al., 2017).
Nevertheless, there may still be indirect associations between the (P)RR and (pro)renin. For instance, Riquier-Brison et al. (2018) observed a link between the (P)RR and renin release, involving prostaglandin E2 (PGE2) generation by COX-2. In line with this finding, macula densa (P)RR conditional knockout (KO) mice displayed diminished plasma renin concentrations and low blood pressure, particularly in the setting of low salt and RAS blockade (Riquier-Brison et al., 2018). Wang et al. (2021) recently confirmed this view. Furthermore, (P)RR ablation is detrimental to kidney development and function (Yosypiv, 2017): nephron progenitor-specific (P)RR deficiency reduced the number of nephrons and resulted in small cystic kidneys (Song et al., 2016), podocyte-specific (P)RR KO led to severe proteinuria and kidney failure (Oshima et al., 2011), and ureteric bud-specific (P)RR deletion induced renal hypodysplasia and defects in urine concentration and acidification capacity (Song et al., 2013). These phenomena suggest that the (P)RR, by interfering with kidney function and fluid homeostasis, is likely to affect JG cell (pro)renin release. Finally, megalin-mediated (pro)renin reabsorption in the proximal tubule involves (P)RR-dependent endosomal acidification (Sun et al., 2020a), as will be discussed below. Taken together, although direct (P)RR-(pro)renin interaction at multiple sites in the body now seems unlikely, the (P)RR and the RAS appear to be indirectly linked at multiple levels.
B. Collecting Duct (Pro)renin and (Pro)renin Receptor
Beyond the JG apparatus, the CD has been proposed as a major second site of (pro)renin synthesis in the kidney (Rohrwasser et al., 1999), particularly under conditions of excess local Ang II such as diabetes and hypertension (Prieto-Carrasquero et al., 2004; Kang et al., 2008). According to this concept, (pro)renin is produced in the principal cells of the CD (Rohrwasser et al., 1999) and secreted in the tubular lumen. It may thus appear in urine (Liu et al., 2011b) along with filtered (pro)renin from the systemic circulation (Roksnoer et al., 2016a). Obviously, to allow prorenin to display activity at this location, either proteolytic cleavage to renin is required or it should undergo a conformational change (Fig. 1). In the absence of any known prorenin-renin converting enzyme in the CD, it has been argued that the (P)RR may help to achieve this conformational change (Nguyen and Muller, 2010). A key finding in support of this notion is that the (P)RR is predominantly localized to the CD, specifically on the luminal membrane of the intercalated cells (Advani et al., 2009). Prorenin synthesized from the principal cells might thus act in a paracrine manner on the intercalated cell (P)RR to generate Ang I, provided that AGT is available and that the local prorenin levels are sufficiently high. Additionally, (pro)renin bound to the (P)RR has been reported to activate signaling pathways such as p38 mitogen-activated protein kinase (MAPK) and extracellular signal-regulated kinase 1/2 (ERK1/2) (Nguyen et al., 2002; Batenburg et al., 2011). A further possibility is that s(P)RR exerts effects of its own (Cousin et al., 2009; Fang et al., 2017; Nakagawa et al., 2017), whereas simultaneously there may be a role for the (P)RR as an accessory protein for vacuolar H+-ATPase (Ludwig et al., 1998) as discussed earlier. Consequently, CD prorenin and the (P)RR may modulate CD cell function through both RAS-dependent and -independent pathways (Fig. 2).
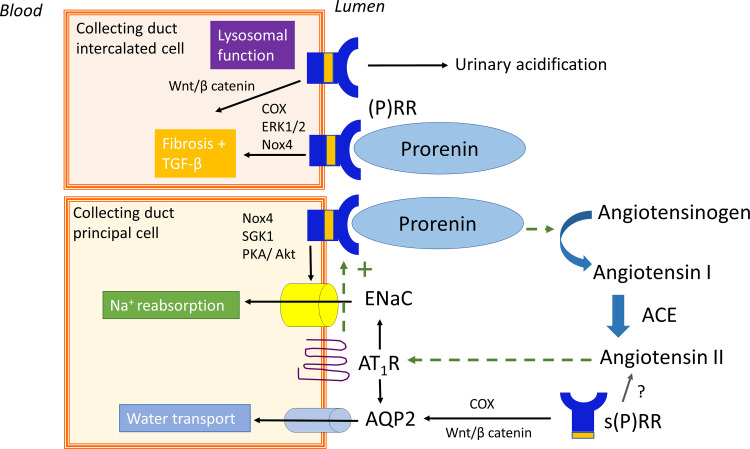
Proposed role of collecting duct prorenin and the (pro)renin receptor [(P)RR]. Dotted lines show the positive feedback loop of angiotensin II-dependent prorenin and (P)RR synthesis. Prorenin binding to the (P)RR has been linked to water transport, sodium reabsorption, and fibrosis. Independent of prorenin, the (P)RR is involved in lysosomal function and fibrosis. Akt, protein kinase B; AQP2, aquaporin 2; Nox4, NADPH oxidase 4; PKA, proteinase kinase A; SGK1, serine/threonine-protein kinase 1.
Several factors have been shown to modulate CD (pro)renin and (P)RR synthesis (Table 1), including Ang II, sodium intake, and hyperglycemia. Under cell culture conditions, Ang II stimulates CD (pro)renin and (P)RR expression via the AT1 receptor (Prieto-Carrasquero et al., 2004; Gonzalez et al., 2011). The Ang II–mediated effects on CD (pro)renin synthesis are contrary to what occurs in the JG apparatus where Ang II inhibits renin synthesis. Similarly, excess systemic Ang II increases renal medullary (P)RR transcript and urinary excretion of the s(P)RR (Gonzalez et al., 2011). Notably, Ang II–stimulated (P)RR expression appears to be mediated via COX-2 through PGE2 and its EP4 receptor (Gonzalez et al., 2014a; Wang et al., 2014). Thus, a feed-forward system might exist within the CD wherein Ang II effects are amplified in the lumen via increased synthesis of CD (pro)renin and/or (P)RR. This mechanism might explain, at least in part, why low sodium intake augments CD (pro)renin and (P)RR expression (Rohrwasser et al., 1999; Quadri and Siragy, 2014). Further, independent of Ang II, sodium depletion has also been shown to modulate CD (P)RR expression via other pathways such as the glycogen synthase kinase 3β/nuclear factor of activated T cell 5/sirtuin-1 pathway and the cGMP/protein kinase G pathways (Quadri and Siragy, 2014). Hyperglycemia is another important regulator of (pro)renin synthesis, and the CD has been suggested to be the origin of the elevated circulating prorenin levels in diabetes (Kang et al., 2008). Simultaneously, circulating renin levels are low in this condition, whereas urinary renin is elevated (Hollenberg et al., 2011; van den Heuvel et al., 2011). Although the latter would be in accordance with CD (pro)renin release in diabetic conditions, a recent study found that the increased urinary renin levels in diabetes were actually due to increased glomerular filtration and impaired proximal tubular reabsorption via megalin (Tang et al., 2019). Moreover, prorenin is usually undetectable in urine, except under conditions where megalin-mediated reabsorption is impaired (van den Heuvel et al., 2011; Roksnoer et al., 2016a). Combined with the fact that Tang et al. (2019) were unable to demonstrate migration of renin lineage cells to the CD, the question arises as to whether local (pro)renin synthesis in vivo truly occurs in the CD (Pohl et al., 2010; Roksnoer et al., 2016a; Sun et al., 2019). An alternative explanation might be that reduced megalin binding of excess filtered prorenin allows its accumulation in the CD under diabetic conditions (Sun et al., 2019; Tang et al., 2019). This may also be true under conditions where Ang II is infused since high Ang II levels will impair glomerular filtration (increasing (pro)renin filtration), whereas Ang II simultaneously downregulates megalin (Hosojima et al., 2009), thus resulting in increased exposure of the CD to filtered (pro)renin.
TABLE 1
(Pro)renin and the (pro)renin receptor [(P)RR] in the collecting duct: synthesis and effects, including effects that involve the soluble s(P)RR
Synthesis | |
---|---|
(Pro)renin | ↑ after Ang II (Prieto-Carrasquero et al., 2004) |
↑ after low sodium (Rohrwasser et al., 1999) | |
↑ after hyperglycemia (Kang et al., 2008) | |
(P)RR | ↑ after Ang II (Gonzalez et al., 2011, 2014b; Wang et al., 2014) |
↑ after low sodium (Quadri and Siragy, 2014) | |
↑ or = after hyperglycemia (Ichihara et al., 2008; Matavelli et al., 2010) | |
Effects (P)RR | |
Water transport | ↑ via Ang II or Ang II–independent (Song et al., 2013; Ramkumar et al., 2015; Lu et al., 2016b; Wang et al., 2016) |
Sodium reabsorption | ↑ partly via Ang II (Ramkumar et al., 2015, 2016b, 2018; Lu et al., 2016a,b; Quadri and Siragy, 2016) |
Fibrosis | ↑ Ang II–independent (Reyes-Martinez et al., 2019) |
Urinary acidification | ↑ (pro)renin-independent (Trepiccione et al., 2016) |
Effects s(P)RR | |
Water transport | ↓ in a polyuria model (Lu et al., 2016b; Wang et al., 2019) |
Sodium reabsorption | ↑ via ENaC (Wang et al., 2020; Feng et al., 2021) |
Blood pressure | ↑ during Ang II infusion (Ramkumar et al., 2021) |
CD (P)RR expression changes with hyperglycemia are inconsistent, with some studies reporting increased (P)RR expression in diabetes (Matavelli et al., 2010) and others finding no difference (Ichihara et al., 2008). A crucial issue remains as to whether the augmented luminal (pro)renin levels under diabetic conditions, derived from either the systemic circulation and/or CD synthesis (Tang et al., 2019), are sufficiently high to allow local angiotensin generation.
A wide range of studies suggest that CD (pro)renin and (P)RR have the ability to modulate CD function (Table 1), including water transport (Ramkumar et al., 2015; Lu et al., 2016b), sodium homeostasis and blood pressure (Lu et al., 2016a; Ramkumar et al., 2016b, 2018), fibrosis (Reyes-Martinez et al., 2019), and acid-base balance (Ludwig et al., 1998; Lu et al., 2013; Daryadel et al., 2016; Trepiccione et al., 2016). Despite initial observations that the effect on water transport involved Ang II–mediated effects related to (P)RR-(pro)renin interaction (Song et al., 2013; Ramkumar et al., 2015; Lu et al., 2016b; Wang et al., 2016), further analyses demonstrated that this was rather due to CD (P)RR effects involving activation of COX and the Wnt/β-catenin pathway (i.e., this was unrelated to Ang II) (Lu et al., 2016b; Wang et al., 2016). Concurrently, CD prorenin acting via the (P)RR can modulate sodium balance via changes in epithelial Na+ channel (ENaC) abundance and activity (Ramkumar et al., 2015; Lu et al., 2016b; Ramkumar et al., 2016b, 2018). These effects have been attributed to NADPH oxidase 4–derived H2O2 (Lu et al., 2016a), serum- and glucocorticoid-regulated kinase-1 (Quadri and Siragy, 2016), and the protein kinase A and protein kinase B pathways (Ramkumar et al., 2016b) and might partly involve Ang II. In accordance with early in vitro studies in VSMCs (Batenburg et al., 2011), prorenin binding to the (P)RR in the kidney has also been suggested to upregulate the release of profibrotic factors like transforming growth factor β1 (TGFβ1) through activation of MAPK/ERK1/2, COX, and NADPH oxidase 4 (Reyes-Martinez et al., 2019). Furthermore, the role of the (P)RR as an accessory protein for vacuolar H+-ATPase (Advani et al., 2009; Lu et al., 2013; Daryadel et al., 2016) needs to be considered since this ATPase is important for urinary acidification, lysosomal function, and autophagy (Trepiccione et al., 2016). Hence, it remains to be determined whether the functional significance of the CD (P)RR goes beyond modulation of vacuolar H+-ATPase (Meima and Danser, 2011).
Finally, recent evidence suggests that the s(P)RR can modulate CD function (Table 1). Recombinant s(P)RR activates Wnt/β-catenin signaling in CD cells and alleviates polyuria in several rodent models with polyuria (Lu et al., 2016b; Wang et al., 2019). Similarly, s(P)RR can regulate CD ENaC function (Wang et al., 2020; Feng et al., 2021) and blood pressure (Feng et al., 2021; Ramkumar et al., 2021). Further, elevated plasma s(P)RR levels have been described in humans and mice with hypertension (Morimoto et al., 2014) and other sodium-retentive states such as heart failure and kidney disease (Fukushima et al., 2013). Conversely, the loss of s(P)RR in mice reduced blood pressure at baseline and decreased Ang II–induced hypertension and kidney injury (Ramkumar et al., 2021). Since the s(P)RR retains the prorenin-binding site, it has been suggested that s(P)RR effects on CD function might involve Ang II signaling (Feng et al., 2021). However, as shown recently, loss of the s(P)RR did not alter the systemic or renal Ang II levels (Ramkumar et al., 2021), in agreement with the observation that the prorenin levels required for such interaction do not occur in vivo. This indicates that s(P)RR acts via RAS-independent mechanisms such as by modulating endothelial cell function (Fu et al., 2021; Ramkumar et al., 2021), and/or pathways within the CD.
C. Liver-Derived AGT Is the Source of Kidney Angiotensins: Importance of Megalin
Because the proximal straight tubule (S3 segment) highly expresses AGT mRNA, local transcriptional activity of the AGT gene was thought to be a major determinant of kidney Ang II generation. Interestingly, Ang II increased renal AGT mRNA via its AT1 receptor, thus potentially creating a feed-forward loop that might contribute to the development of diabetic kidney disease (DKD), also because high glucose similarly increases renal AGT mRNA (Gonzalez-Villalobos et al., 2008; Nishiyama et al., 2008; Nishiyama and Kobori, 2018). However, studies using tissue-specific Agt KO mice demonstrated that the liver is the major source not only of plasma AGT but also renal AGT. Indeed, liver-specific Agt KO decreased plasma AGT by more than 95%, and similar decreases were seen for AGT and Ang II in the kidney (Matsusaka et al., 2012). Recent studies making use of hepatocyte-directed small interfering RNA (siRNA) for AGT in rats displaying hypertension and renal injury [spontaneously hypertensive rats (SHRs), deoxycorticosterone acetate-salt-treated hypertensive rats, and five-sixths nephrectomy rats], or liver-targeted AGT antisense oligonucleotides in normotensive cynomolgus monkeys yielded the same conclusions (Uijl et al., 2019, 2021; Bovée et al., 2021; Kukida et al., 2021).
In contrast, proximal tubule–specific Agt KO did not affect renal AGT and Ang II content, nor kidney histology, blood pressure, and urinary sodium excretion (Matsusaka et al., 2012). Urinary AGT protein was decreased by approximately 50% in proximal tubule–specific Agt KO mice, indicating that AGT protein produced in the S3 segment is directly excreted into urine. It should be noted that lack of an abnormal basal gross phenotype does not mean lack of functional significance because there may be compensation by other factors. In addition, effects of Ang II infusion or diabetes have not been tested in these mice. Ramkumar et al. (2016a) reported that nephron-specific Agt KO mice showed lower blood pressure, but since in these mice the Agt gene was also disrupted in the liver, this phenotype likely represents combined hepatic and renal AGT suppression.
Immunostaining detected AGT protein in proximal convoluted tubules (S1 and S2 segments), whereas AGT mRNA is detected in proximal straight tubules (S3 segment). AGT is stained in a granular pattern, similarly to that of albumin. This indicates that AGT in the S1 and S2 segments, like albumin, is reabsorbed from tubular fluid. Indeed, a great number of proteins, including albumin, is reabsorbed in the proximal tubules via the multiligand receptor megalin. Megalin KO mice revealed that AGT staining was dependent on megalin (Pohl et al., 2010). For unknown reasons, the glomerular permeability of AGT is approximately one-fourth that of albumin (Nakano et al., 2012) despite the fact that the molecular mass of AGT (53 kDa) is lower than that of albumin (66 kDa) and lacks negative charge. Nevertheless, the above findings indicate that a small amount of plasma AGT is filtered through the glomerulus and reabsorbed by the proximal convoluted tubule (S1 and S2 segments) via megalin. Data from humans displaying megalin dysfunction fully concur with this concept (Roksnoer et al., 2016a).
This notion leads to the possibility that disruption of the glomerular filtration barrier increases delivery of AGT to the tubules and might thus enhance kidney Ang II generation. In fact, when the glomerular barrier was disrupted by inducing podocyte injury, the amount of reabsorbed AGT was markedly increased, concurrently with an increase in urinary AGT (Matsusaka et al., 2012). Importantly, the renal Ang II content was also increased, independently of renal renin. In accordance with this finding, Matsuyama et al. (2021) showed that the renal Ang II levels correlated with the glomerular filtration of AGT in doxorubicin (Adriamycin) nephropathy rats. Studies using tissue-specific Agt KO mice again showed that the increased renal Ang II generation by podocyte injury was completely dependent on liver-derived AGT (Matsusaka et al., 2014). In addition, ureteral obstruction attenuated the increase of AGT protein and Ang II in kidneys with podocyte injury (Okabe et al., 2015), indicating that the major source of increased renal Ang II in podocyte injury is filtered AGT. Although podocyte injury also increased AGT mRNA in proximal tubular cells, this did not contribute to renal Ang II because proximal tubule–specific Agt KO did not decrease renal Ang II.
Earlier studies showed that infusion of Ang II into rodents increased renal AGT mRNA, renal and urinary AGT protein, and renal Ang II content (Navar et al., 2002; Kobori et al., 2007b; Gonzalez-Villalobos et al., 2008). The increase in renal AGT mRNA was thought to be the cause of the increase in renal Ang II. However, as discussed above, the contribution of renal AGT to renal Ang II synthesis, both under control and nephrotic conditions, is virtually absent. Tissue-specific Agt KO mice are needed to verify whether renal AGT contributes to renal Ang II synthesis after Ang II infusion. A possible alternative mechanism is that Ang II increases glomerular capillary pressure, which may increase glomerular filtration of plasma AGT and tubular reabsorption of AGT. Supporting this hypothesis, it was shown that Ang II infusion increased granular staining of AGT in S1 and S2 segments (Gonzalez-Villalobos et al., 2008).
The above results suggest that AGT protein reabsorbed via megalin contributes to Ang II generation at renal tissue sites. This hypothesis was tested using proximal tubule–specific megalin KO mice (Koizumi et al., 2019). As expected, renal AGT protein was markedly decreased and urinary AGT excretion was increased in these mice. However, their intrarenal Ang II content was comparable to that in control mice, without changes in blood pressure and urinary sodium excretion. This implies that the AGT reabsorbed by the proximal tubular cells via megalin in healthy, normotensive mice does not contribute to local Ang II generation (Fig. 3). Therefore, under normal circumstances, the major source of renal Ang II is probably AGT within the capillary lumen or interstitium of the kidney. Indeed, diffusion of AGT from blood to the interstitial space is known to occur (de Lannoy et al., 1997). In contrast, Ye et al. (2019) reported that suppression of megalin by antisense oligonucleotides decreased renal Ang II by 70% in mice without podocyte injury. A unifying concept might be that in Koizumi’s study, renal renin was sufficiently upregulated to restore renal Ang II, whereas in Ye’s study it was not (Sun et al., 2020b). If true, this would imply that reabsorbed AGT does contribute after all to renal Ang II generation under normal conditions. However, neither study reported renal renin levels, and thus this question remains to be answered.
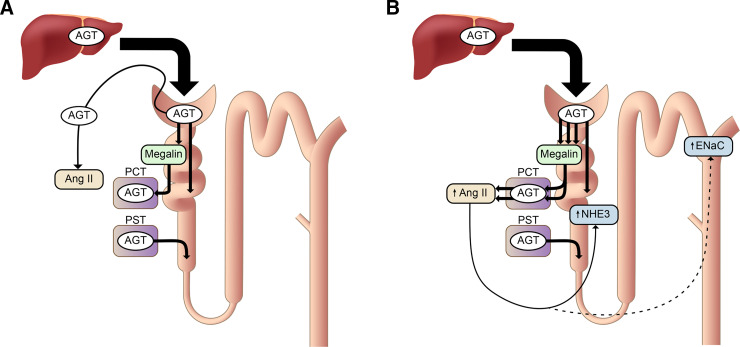
Proposed mechanism of renal angiotensin (Ang) II generation and its function. (A) Under normal conditions, a portion of plasma AGT is filtered through the glomeruli and reabsorbed by proximal convoluted tubules (PCTs, S1, and S2 segments) via megalin. Its contribution to renal Ang II generation is marginal. AGT is synthesized in proximal straight tubules (PSTs, S3 segment), but this AGT also makes little contribution to renal Ang II formation, although it may appear in urine. Renal Ang II is probably generated from liver-derived AGT in the capillary lumen or interstitium. (B) In nephrotic syndrome, podocyte injury increases glomerular leakage of plasma AGT. The filtered AGT is reabsorbed by proximal tubules via megalin and thereafter converted to Ang II. The increased renal Ang II content may contribute to sodium retention, possibly by activating Na+/H+ exchanger 3 (NHE3) and the epithelial Na+ channel (ENaC) (Koizumi et al., 2019).
When podocytes are severely injured, a large amount of plasma AGT leaks into the tubular lumen. This AGT will be avidly reabsorbed by proximal tubular cells via megalin. In this situation, megalin KO further increased urinary AGT as expected (Koizumi et al., 2019). The kidneys of these animals showed massive proteinaceous casts. Immunostaining revealed that most AGT staining occurred on these proteinaceous casts, whereas proximal tubular cells were devoid of staining of AGT. The renal Ang II content in the megalin KO mice with podocyte injury was significantly reduced. These results indicate that, in the nephrotic state, AGT filtered through the glomerulus and reabsorbed via megalin by the proximal tubule is converted to Ang II (Fig. 3), whereas AGT in the tubular lumen is not.
The suppression in renal Ang II was paralleled by a reduction in renal sodium reabsorption in megalin KO mice with podocyte injury. Podocyte injury does not increase blood pressure but markedly increases urinary sodium reabsorption, which causes retention of extracellular fluid and edema formation, two hallmarks of the nephrotic syndrome. In megalin KO mice with podocyte injury, this increase in urinary sodium reabsorption was less prominent. In addition, the cleaved forms of α- and γ-subunits of ENaC, which reflect the activation of this channel, and membrane-bound Na+/H+ exchanger 3 (NHE3) were increased in megalin-intact nephrotic mice, and this increase was significantly attenuated in nephrotic megalin KO mice (Koizumi et al., 2019). Collectively, these data suggest that increased renal Ang II by podocyte injury may contribute to sodium retention by activating ENaC and NHE3. The renal Ang II generation from the leaked plasma AGT may be a mechanism underlying the overfill hypothesis of nephrotic syndrome (i.e., proteinuria primarily stimulates sodium reabsorption).
It should be noted, however, that these data were obtained in an extreme condition, where a very severe nephrotic syndrome was rapidly developing. Translating the role of megalin in renal Ang II generation to all proteinuric conditions is therefore not yet possible. In this regard, it was reported that megalin KO mice with mild proteinuria show more activated α-subunit of ENaC, without changes in renin and aldosterone (Kastner et al., 2009). This opposes the findings of the observations in megalin KO mice with severe nephrotic syndrome. It might involve ENaC activation by proteases in urine, although this was described for the γ-subunit of ENaC only (Hinrichs et al., 2020). If confirmed, however, sodium reabsorption in nephrotic syndrome and other proteinuric conditions might be treated with an ENaC blocker (amiloride) rather than RAS blockers.
The next obvious question is where and how the reabsorbed AGT is converted to Ang II. Based on in vitro data in cultured opossum kidney cells, Pohl et al. (2010) reported that a portion of AGT is transcytosed to the basolateral membrane and released into the interstitium. Renin has a smaller molecular mass (43 kDa) and a larger glomerular sieving coefficient than AGT, whereas its glomerular leakage is similarly increased after podocyte injury (Roksnoer et al., 2016a). As discussed, renin is also reabsorbed via megalin, and this is true for prorenin as well (Sun et al., 2020a). Taken together, these data suggest that Ang II is likely formed in proximal tubules, although the exact location of this formation (tubular lumen or peritubular interstitium) still needs to be identified. Future studies should also investigate how hepatic AGT ensures local generation of Ang II at the level of the CD, which is an important site for controlling sodium reabsorption in nephrotic syndrome. Given the well known beneficial effects of RAS blockers on tubulointerstitial injury, it now seems likely that this relies on interference with Ang II generated via a megalin-dependent mechanism. This may offer new insights for the treatment of this condition.
D. ACE, Chymase, and the Site of Angiotensin Generation
Renal ACE is abundantly expressed in the brush border of the proximal tubule (apical and basolateral) but can also be found in vascular endothelial cells, mesangial cells, and distal nephron segments (Metzger et al., 1999; Gonzalez-Villalobos et al., 2013). ACE has been reviewed before in this journal (Bernstein et al., 2012). It is a peptidyl dipeptidase with two catalytic domains (N and C), of which the C-domain is responsible for Ang I-II conversion (van Esch et al., 2005; Arendse et al., 2019). Classically, ACE is the enzyme responsible for Ang II formation from Ang I. The renoprotective effects of ACE inhibitors (ACEIs) demonstrated in multiple clinical trials are in full agreement with this concept. Both the human and rat glomerular vascular beds are remarkably devoid of endothelial ACE compared with other renal and extrarenal vascular beds (Metzger et al., 2011). This is believed to afford protection against excess Ang II–mediated renal vasoconstriction, keeping renal blood flow high.
Recently, chymase, discovered more than 30 years ago in human heart homogenates (Urata et al., 1990), has been put forward as a major Ang I-II converting enzyme in the kidney (Park et al., 2010; Kaltenecker et al., 2020). A major reason for invoking additional converting enzymes is the so-called Ang II escape (i.e., the return of renal Ang II levels to baseline levels during prolonged ACE inhibition). If true, one would expect the clinical effectiveness of AT1 receptor blockers (ARBs) to be better than that of ACEIs, although in reality they are similar (Wu et al., 2013; Thomopoulos et al., 2017). Moreover, renal Ang II levels are negligible in ACE KO animals (Alexiou et al., 2005), and chymase inhibition was ineffective in patients with DKD (Rossing et al., 2021). Here it is important to stress that chymase is largely located intracellularly and thus normally may not ‘see’ Ang I, except when tissue is homogenized (Kaltenecker et al., 2020). A much more logical reason for the return of renal Ang II levels is therefore that ACE inhibition will result in a rapid renin rise due to the drop in Ang II. Additional counterregulation may occur via the upregulation of ACE. However, such renal ACE upregulation is usually of modest proportion (2- to 3-fold) (Gonzalez-Villalobos et al., 2013) compared with the many 100-fold upregulation in renin levels that the body is capable of by recruiting additional renin-synthesizing cells (Balcarek et al., 2014). For instance, in case of 90% ACE inhibition, a 10-fold rise in renin is sufficient to allow Ang II levels to return to normal, whereas a 2-fold rise in ACE would have negligible consequences.
Early studies making use of infusions of 125I-labeled angiotensins (i.e., allowing the detection of 125I-angiotensins at levels that do not affect blood pressure) revealed that there is substantial uptake of circulating 125I-Ang II at renal tissue sites: its steady state tissue levels corresponded to ≈4 times the steady-state plasma levels of 125I-Ang II (van Kats et al., 2001a; Zhuo et al., 2002). Uptake depended entirely on binding to AT1 receptor (van Kats et al., 1997; Zhuo et al., 2002). At the same time, the levels of endogenous Ang II at renal tissue sites were up to 100 times higher than the plasma levels of endogenous Ang II, and thus it could be calculated that, despite significant uptake of circulating Ang II, the majority (>95%) of renal Ang II had been synthesized locally by renal ACE (Schalekamp and Danser, 2006a,b). Infusion studies with 125I-Ang I confirmed that this was not due to the conversion of plasma-derived Ang I. In other words, these studies unequivocally proved that both Ang I and Ang II are produced locally at renal tissue sites and that renal Ang II is derived from locally generated Ang I and not from plasma-derived Ang I. Furthermore, although most, if not all, renal Ang II was cell associated (i.e., either bound to membrane AT1 receptor or located intracellularly) (van Kats et al., 2001b), this was not due to intracellular angiotensin generation but the consequence of AT1 receptor binding followed by Ang II–AT1 receptor internalization. Indeed, there was no cell-associated Ang II in AT receptor KO mice, despite the tremendous rises in renin that occur in such animals (van Esch et al., 2010b). Therefore, renal angiotensin production occurs extracellularly, in the renal interstitial space, and/or on the surface of renal cells.
E. ACE2, Neprilysin, and Other Angiotensinases
In addition to the classic cascade resulting in the formation of the octapeptide Ang II [=Ang-(1-8)] from the decapeptide Ang I [=Ang-(1-10)] by ACE, ACE2 can cleave Ang I to Ang-(1-9), which is then converted to Ang-(1-7) by ACE (Fig. 4). Alternatively, Ang-(1-7) can be formed directly from Ang I by neprilysin (Domenig et al., 2016). Ang II degradation involves aminopeptidase A (APA), neprilysin, dipeptidyl aminopeptidase III (DDP), aspartate decarboxylase, and neurolysin (McDonald et al., 1974; Brown et al., 2001; Rice et al., 2004; Marahrens et al., 2021). In the kidney, neprilysin and APA are the two most relevant Ang II–degrading enzymes. DDP generates Ang IV [=Ang-(3-8)] by cleaving the N-terminal dipeptide from Ang II and can then further cleave Ang IV (Pang et al., 2016). DDP has a much higher substrate affinity for Ang IV than for Ang II and also cleaves Ang-(1-7), but its importance for Ang II degradation within the kidney is limited. Alternatively, Ang IV can also be formed from Ang III [=Ang-(2-8)] by aminopeptidase N (Wysocki et al., 2015). Aspartate decarboxylase decarboxylates the N-terminal amino acid of Ang II (aspartic acid) to form Ang A (Jankowski et al., 2007). Ang A therefore only differs from Ang II in its first amino acid (alanine instead of aspartic acid). ACE2 can hydrolyze Ang A to form alamandine (Lautner et al., 2013). Neurolysin cleaves Ang II to Ang-(1-4) and Ang-(5-8) (Brown et al., 2001).
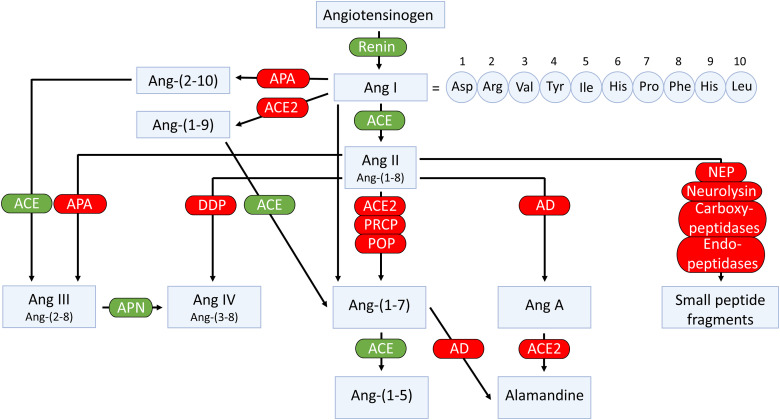
Enzymatic pathways involved in the generation and metabolism of angiotensins. AD, aspartate decarboxylase.
APA degrades Ang II to Ang III by cleaving its N-terminal amino acid. Additionally, APA converts Ang I to Ang-(2-10), which can then be converted to Ang III by ACE (Wysocki et al., 2015). Mice with a genetic deficiency of APA have increased blood pressure, possibly as a result of increased levels of Ang II (Mitsui et al., 2003; Marahrens et al., 2021). Within the kidney, strong expression of APA was found in the glomerulus and to a lesser extent in tubular structures. Immunohistochemistry observed APA costaining with the podocyte marker podocin. In isolated mouse glomeruli, the APA inhibitor amastatin reduced the degradation of Ang II, whereas the ACE2 inhibitor MLN-4760 did not (Marahrens et al., 2021). This suggests that at the glomerular level, Ang II degradation is due to APA rather than ACE2. Indeed, glomerular Ang II levels in APA KO mice were three times higher than in wild-type mice. Remarkably, APA KO reduced renal ACE, most likely to prevent excessive Ang II accumulation. Of interest, knoblike structures in the glomerular basement membrane of APA deficient mice were observed, and when challenged with Ang II, they rapidly developed albuminuria, suggesting an important role for APA in glomerular structure and function, likely exerted via the regulation of the metabolism of Ang II within the glomerulus.
Neprilysin, also known as neutral endopeptidase, degrades both Ang I and II (Rice et al., 2004). Immunohistochemistry of human kidney biopsies revealed strong neprilysin expression in glomerular epithelial cells (Domenig et al., 2016). Studies using the neprilysin inhibitor thiorphan in mouse and human kidney homogenates led to a marked reduction of Ang-(1-7) formation from Ang I (Domenig et al., 2016). Although this suggests that neprilysin is crucial for Ang-(1-7) formation at renal tissue sites, neprilysin inhibition in rats did not alter renal Ang-(1-7) levels (Roksnoer et al., 2015). In 2-kidney, 1-clip Goldblatt hypertensive mice, neprilysin was markedly reduced in the clipped kidney (Alawi et al., 2021), whereas in the kidney of diabetic db/db mice (a type 2 diabetes model), neprilysin was also significantly decreased (Alawi et al., 2020).
There are three enzymes that cleave the C-terminal amino acid (phenylalanine) of Ang II to generate Ang-(1-7): prolyloligopeptidase (POP), also known as prolylendopeptidase, prolylcarboxypeptidase (PRCP), and ACE2 (Donoghue et al., 2000; Tipnis et al., 2000; Maier et al., 2017; Serfozo et al., 2020). The latter has received the most attention, and it is indeed the most potent of the three.
POP is a serine protease that preferably hydrolyzes proline-containing peptides at the carboxyl end of proline residues (Serfozo et al., 2020). POP is preferentially an intracellular enzyme, and its contribution to renal Ang II–Ang-(1-7) conversion is modest compared with ACE2 (Serfozo et al., 2020). Indeed, in kidneys of POP KO mice, Ang-(1-7) formation from Ang II was unaltered (Grobe et al., 2013; Serfozo et al., 2020), although one study in mouse homogenates reported that POP was the main enzyme forming Ang-(1-7) from Ang II (Domenig et al., 2016). The reason for this discrepancy is unclear, but all studies agree that in the human kidney ACE2 is the dominant Ang-(1-7)–forming enzyme (Domenig et al., 2016; Grobe et al., 2013; Serfozo et al., 2020). POP KO mice have no detectable plasma Ang-(1-7) levels, suggesting that Ang-(1-7) in the circulation relies on POP (Serfozo et al., 2020).
PRCP is a serine carboxyprotease cleaving the C-terminal amino acid of various peptides where the penultimate amino acid is proline (Odya et al., 1978). It cleaves both Ang II and III. PRCP, first reported as a soluble lysosomal enzyme, can also be found extracellularly, either membrane bound or in soluble form (Jeong and Diano, 2013). In mice, PRCP deficiency increases blood pressure and results in left ventricular hypertrophy (Maier et al., 2017). Simultaneously, moderate glomerular hypertrophy and mesangial expansion occurred, without alterations in glomerular filtration rate (GFR). In 2-kidney, 1-clip Goldblatt hypertensive mice, PRCP was markedly reduced (Grobe et al., 2015).
ACE2 shares 42% homology with the metalloprotease catalytic domains of ACE (Donoghue et al., 2000; Tipnis et al., 2000). Yet, although the peptidyl dipeptidase ACE removes C-terminal dipeptides from its substrates, ACE2 functions as a monocarboxypeptidase. Ang-(1-7) can be further cleaved by ACE to form Ang-(1-5) (Rice et al., 2004). ACE2 is a zinc metalloprotease that in its full-length form has 805 amino acids and is anchored to the cell membrane by a short transmembrane domain (Turner and Hooper, 2004). The full-length membrane-bound ACE2 is expressed at high levels in multiple tissues, including kidney, testes, intestine, and heart (Donoghue et al., 2000; Tipnis et al., 2000). At very low levels it is also expressed in lung, where it is restricted to apical epithelial and ciliated cells in the upper respiratory tract and to type 2 pneumocytes in the lower respiratory tract (Serfozo et al., 2020; Ziegler et al., 2020). The importance of the location of ACE2 protein in these alveolar cells stems from it being the main cell entry receptor for severe acute respiratory syndrome coronavirus 2 (SARS-CoV-2) (Wan et al., 2020).
The soluble form of ACE2, which lacks the transmembrane domain, has 740 amino acids (Vickers et al., 2002). This soluble form is enzymatically active and is shed into blood, urine, and cerebrospinal fluid by ADAM-17 (Lambert et al., 2005). ACE2 can be also cleaved by mechanisms distinct from ADAM-17 that do not lead to the formation of the enzymatically active soluble ACE2. Specifically, transmembrane serine protease 2 (TMPRSS2), human airway trypsin-like protease, and hepsin have been reported to cleave ACE2 and to form short C-terminal fragments of about 15 kDa (Shulla et al., 2011; Heurich et al., 2014).
Within the normal kidney, full-length ACE2 is expressed abundantly in the apical membrane of the proximal tubule (Ye et al., 2004, 2006). In mice, ACE2 is also present in glomerular parietal cells and to a lesser extent in visceral epithelial cells (podocytes) (Ye et al., 2004, 2006). These findings are in agreement with human data derived from immunohistochemistry, immunofluorescence, and single cell analysis showing clear detection of ACE2 in proximal tubules and to a lesser extent in parietal epithelial cells of Bowman’s capsule (Lely et al., 2004). In human kidney homogenates, ACE2 is the main contributor to the formation of Ang-(1-7) from Ang II (Domenig et al., 2016; Serfozo et al., 2020). Alterations in kidney ACE2 can therefore affect the balance of these two peptides and may thus contribute to diabetic and hypertensive kidney disease. In diabetes, the expression of ACE2 seems to differ at the tubular and glomerular level (Lores et al., 2020). In db/db mice, glomerular ACE2 expression is decreased, whereas tubular ACE2 expression is increased (Ye et al., 2004, 2006). Similarly, in young streptozotocin (STZ)-treated diabetic rats (a type 1 diabetes model), glomerular ACE2 activity was also decreased (Leehey et al., 2008). Studies in humans with DKD confirm this pattern (Mizuiri et al., 2008; Reich et al., 2008). Kidney biopsies from patients with type 2 diabetes examined by immunohistochemistry or reverse-transcription polymerase chain reaction (RT-PCR) also showed decreased glomerular ACE2 staining/mRNA levels compared with healthy controls or controls that had focal segmental glomerulosclerosis or chronic allograft nephropathy. In these studies, however, tubular ACE2 was also found to be decreased. Both studies found glomerular ACE expression to be increased in diabetic patients.
In experimental rat models of hypertension, renal ACE2 expression is decreased (Crackower et al., 2002). These findings were confirmed in adult SHRs (Zhong et al., 2004). Increased ACE2 levels, by contrast, were found in tubules from young SHRs prior to the onset of hypertension, which then declined with the onset of hypertension (Tikellis et al., 2006). In the 2-kidney, 1-clip mouse model of hypertension, ACE2 and neprilysin expression decreased in parallel (Alawi et al., 2021). Similar to the data in animal models, biopsies from patients with hypertensive nephropathy examined by immunohistochemistry show a marked decrease of ACE2 staining in tubules (Koka et al., 2008). An immunohistochemistry study in patients with hypertensive nephrosclerosis also found tubulointerstitial ACE2 levels to be decreased, whereas glomerular ACE2 expression was not altered as compared with controls (Wang et al., 2011). In mice that underwent renal ischemia reperfusion injury, immunohistochemistry revealed reduced ACE2 staining in the corticomedullary area (Fang et al., 2013). In an animal model of ischemia reperfusion injury-induced acute kidney injury (AKI), moreover, decreased ACE2 levels were found 24 and 48 hours postsurgery (Shirazi et al., 2019).
The effect of ACE2 deficiency on kidney injury has been studied in a model of genetic ACE2 ablation. Ang II infusion in this model resulted in higher levels of blood pressure than in control mice (Gurley et al., 2006). In a model of diabetic kidney injury, ACE2 KO mice displayed increased urinary albumin excretion and more severe histopathology (Wong et al., 2007). Wysocki et al. (2014) found increased markers of oxidative stress in the kidneys of ACE2 KO mice at baseline conditions. Also, in the ischemia reperfusion model of kidney injury, ACE2 KO mice showed increased markers of oxidative stress and proinflammatory cytokines, combined with increased inflammatory cell infiltration (Fang et al., 2013). Yang et al. (2012b) studied the hindlimb-ischemia reperfusion model and found that ACE2 KO mice had more severe kidney injury than controls.
In summary, ACE2 deficiency worsens the consequences of kidney injury, whereas several kidney disease models also display lowered kidney ACE2 levels. Based on this, intact ACE2 expression is often seen as a protective factor. However, given the many substrates of ACE2, whether this relates exclusively to a disturbed Ang-(1-7)/Ang II balance remains to be proven.
F. Angiotensin Levels in the Kidney
Measuring angiotensins is notoriously difficult, and consequently angiotensin levels reported in the literature vary widely, often by many orders of magnitude. Obtaining blood samples for angiotensin quantification requires the immediate addition of a protease inhibitor cocktail, assuring efficient stabilization of angiotensin metabolites by instantly blocking all proteases involved in their metabolism. The sampling time has to be kept as short as possible, and an immediate and efficient mixture of the blood sample and the inhibitor cocktail is essential to avoid artifacts, which are particularly challenging when collecting blood samples from mice. The importance of the sampling time is often underestimated and is likely the cause of unexpected peptide shifts despite the use of inhibitor cocktails. Tissue samples cannot be easily mixed with an inhibitor cocktail and should thus be snap-frozen in liquid nitrogen and stored at −80°C until analysis. The actual quantification often relies on commercial radioimmunoassays or ELISAs that have not been validated thoroughly and hence yield results that are entirely unreliable, particularly when using tissue homogenates with a lot of background noise (Chappell et al., 2021). Instead, prior high-performance liquid chromatography separation or liquid chromatography-tandem mass-spectrometry should be applied. Table 2 summarizes data in untreated rats where these methods were used and where a range of metabolites in appropriately collected samples was determined (Campbell et al., 1991, 1995c; Roksnoer et al., 2015; Uijl et al., 2019, 2021).
TABLE 2
Levels of angiotensin metabolites in kidney and blood in various rat strains
SD | (mRen2)27 | SD | SHR | (mRen2)27 | |
---|---|---|---|---|---|
n = 6 mean ± S.E.M. | n = 8 mean ± S.E.M. | n = 6–8 mean (IQL range) | n = 8 mean ± S.D. | n = 5–8 mean ± S.E.M. | |
Kidney (fmol/g) | |||||
Ang-(1-10) | 234 ± 33 | 20 ± 2 | 564 (313–907) | 413 ± 139 | 163 ± 69 |
Ang-(1-8) | 338 ± 33 | 474 ± 40 | 729 (431–1892) | 431 ± 44 | 388 ± 36 |
Ang-(1-7) | 33 ± 8 | ND | 173 (65–279) | 66 ± 45 | 20 ± 3 |
Ang-(1-5) | ND | ND | 17 (<13–40) | 16 ± 6 | 13 ± 4 |
Ang-(2-8) | <15 | ND | 75 (44–121) | <8 | 26 ± 3 |
Ang-(3-8) | ND | ND | <10 | ND | 5 ± 0 |
Ang-(2-10) | 37 ± 5 | ND | ND | ND | 30 ± 15 |
Ang-(1-9) | 113 ± 19 | ND | ND | ND | 42 ± 12 |
Blood (fmol/ml) | |||||
Ang-(1-10) | 100 ± 35 | 78 ± 5 | 141 (103–200) | 375 ± 196 | ND |
Ang-(1-8) | 47 ± 15 | 125 ± 9 | 105 (75–141) | 36 ± 36 | ND |
Ang-(1-7) | 5 ± 2 | 13 ± 1 | <8 | <7 | ND |
Ang-(1-5) | ND | ND | 5 (3–6) | 2 ± 1 | ND |
Ang-(2-8) | 29 ± 8 | ND | 4 (<2.5–6) | <3 | ND |
Ang-(3-8) | ND | ND | 4 (3–6) | 4 ± 6 | ND |
Ang-(2-10) | 34 ± 12 | ND | ND | ND | ND |
Ang-(1-9) | <1 | ND | ND | ND | ND |
IQL, interquartile; m(Ren2)27, transgenic rat overexpressing mouse Ren2 gene; ND, not done; SD, Sprague Dawley.
Data are from Campbell et al. (1991, 1995b); Roksnoer et al. (2015); and Uijl et al. (2019, 2021) and have been determined either by radioimmunoassay after high-performance liquid chromatography separation (first two columns) or by using liquid chromatography-tandem mass-spectrometry (last three columns).
These data show that in general, Ang I and II are the main metabolites in blood, with Ang I levels usually being several-fold higher than those of Ang II. The blood levels of Ang-(1-7) and Ang III are 5- to 10-fold below those of Ang II and often below detection limit. In the kidney, Ang II is the main metabolite, with levels in the order of several 100 fmol/g (i.e., easily 10- to 20-fold higher than its levels in blood). Tissue Ang-(1-7) and Ang III levels are 5- to 10-fold lower than the tissue levels of Ang II, comparable to what is observed in blood. Assuming that Ang I has no role (i.e., that it does not bind to any of the known AT receptors), these data imply that Ang II is by far the most important active angiotensin metabolite in both blood and tissue, with only minor roles for Ang-(1-7) and Ang III. Importantly, and perhaps not surprising, angiotensin levels in other species (mice, pigs, humans), if measured appropriately, are remarkably similar to those in rats (Lawrence et al., 1990; van Kats et al., 2001a; Alexiou et al., 2005; Fisher et al., 2008; Balcarek et al., 2014). This should be the case since the affinity of Ang II for its receptors is identical in all species, and thus it would be hard to explain why tissue Ang II levels in one species would be, for instance, 10- or 100-fold higher than in another species. Moreover, no major differences in Ang I and II levels were observed between renal medulla and cortex (van Kats et al., 2001a).
Recent studies have introduced the concept of ‘equilibrium levels’ of angiotensins in serum or plasma (Pavo et al., 2021). These are obtained by incubating a sample at 37°C in the absence of inhibitors. This opposes the conditions of a plasma renin activity measurement, where plasma is incubated for a fixed amount of time in the presence of a cocktail of angiotensinase inhibitors that prevent the breakdown of Ang I (Campbell et al., 2009). Thus, only Ang I is generated under the latter assay conditions, and the amount of Ang I generated per hour is independent of the incubation period. Data are often expressed as ng Ang I per ml per hour, with Ang I generation being linear over 24 hours. Blood contains renin, AGT, ACE, and a great variety of angiotensinases. Thus, when incubating plasma or serum at 37°C, all enzymes will act simultaneously, yielding virtually every angiotensin metabolite that has been described. Here it is important to realize that this situation also exists in vivo, with one exception: ACE and all angiotensinases occur at much higher levels on endothelial cells (and potentially other vascular cells), resulting in an entirely different metabolism pattern. Indeed, the levels of ACE and ACE2 in blood represent at most a few percent of the amount that is cell surface bound, and when additionally considering blood flow (allowing exposure to the entire vascular bed in a matter of minutes), their role is negligible. Hence, the angiotensin half-life in vivo is in the order of seconds (Danser et al., 1992), whereas in isolated plasma/serum it is easily several orders of magnitude longer (van Esch et al., 2005). Moreover, it is questionable whether the ratio at which all enzymes occur in serum or plasma is identical to that in the in vivo situation. As a consequence, incubating plasma/serum will generate ‘equilibrium levels’ (given the fact that they are obtained after an incubation period, they do not really represent levels and should, like plasma renin activity, be expressed per time component) that have little to do with the in vivo situation, and quite often metabolites occur that are not seen in vivo. Thus, the equilibrium approach might provide an insight on what enzymes are present in serum or plasma but cannot be used to draw conclusions on the in vivo levels of angiotensin metabolites.
III. Receptors Involved in the Effects of Kidney Angiotensins
A. AT1 Receptor
Ang II, Ang III, and Ang-(1-7) have all been reported to play diverse and important roles in the regulation of renal hemodynamics and tubular transport activities via activation of their respective receptors or receptor binding sites (Kobori et al., 2007b; Forrester et al., 2018; Li et al., 2018b). Ang II is the major effector of all angiotensin peptides in the kidney. Indeed, it reduces renal blood flow and determines glomerular filtration by contracting efferent arterioles more strongly than afferent arterioles. In addition, it regulates fibrosis and inflammation and stimulates sodium and bicarbonate (HCO3−) reabsorption via activation of NHE3, the basolateral Na+-HCO3− cotransporter and basolateral Na+/K+-ATPase (Kobori et al., 2007b; Forrester et al., 2018; Li et al., 2018b). Finally, as has been discussed before (in section II.B), it stimulates ENaC. These well recognized effects of Ang II in the kidney are mediated primarily by AT1 receptors (Forrester et al., 2018; Kobori et al., 2007b), whereas the AT2 receptor may counteract some or all of these effects (Siragy et al., 1999; Kemp et al., 2016; Li et al., 2020). The molecular structure, pharmacological classification, and signaling transduction pathways have been well characterized and comprehensively reviewed elsewhere (Gasparo et al., 2000; Kobori et al., 2007b; Forrester et al., 2018). Signaling involves both G protein–coupled kinases and β-arrestins. The latter were originally believed to mediate receptor internalization and desensitization but now are also known to couple directly to MAPK, ERK1/2, and nuclear factor-kappa B (NF-κB) (Violin et al., 2013). Intriguingly, this new insight has led to the development of β-arrestin–biased AT1 receptor agonists (e.g., TRV027, SII, and TRV120023), which are being evaluated in heart failure (Pang et al., 2017), based on the concept that such drugs would not only act as an ARB (reducing blood pressure, improving renal blood flow, and enhancing sodium excretion) but would also increase cardiac contractility via the β-arrestin pathway (Boerrigter et al., 2012; Violin et al., 2013). Yet, Wang et al. (2017c) reported that the AT1 receptor–β-arrestin–ERK1/2 signaling pathway is responsible for the development of renal fibrosis, whereas Carneiro de Morais et al. (2015) observed that TRV120023 inhibits NHE3 in opossum proximal tubule cells. Thus, whether such drugs would be useful tools in kidney disease is still unclear.
In rodents, the AT1 receptor is divided into two subtypes, AT1a and AT1b (Murphy et al., 1991), to which Ang II binds with high specificity and affinity. Interestingly, only one AT1 receptor gene is identified in humans. AT1a receptors are coupled to Gq/11 proteins and activation of phospholipase C (Gasparo et al., 2000; Kobori et al., 2007b; Forrester et al., 2018). This leads to the generation of inositol triphosphate and diacylglycerol, with subsequent mobilization of intracellular Ca2+ and activation of protein kinase C. AT1 (AT1a) receptor–mediated increases in intracellular Ca2+ are associated with well recognized contraction of VSMC and vasoconstriction of blood vessels, whereas activation of protein kinase C triggers diverse downstream signaling transduction pathways that mediate long-term genomic and transcriptional effects such as oxidative stress responses, cellular growth, transporter expression (NHE3, Na+/K+-ATPase, and the Na+/HCO3− cotransporter), tissue fibrosis, and target tissue injury (Li and Zhuo, 2011; Crowley and Rudemiller, 2017; Li et al., 2018b). The pharmacological characteristics of AT1b receptors remain poorly investigated.
AT1 receptors belong to the AT1a subtype in most, if not all, target tissues, whereas AT1b receptor expression is restricted to a limited number of target tissues. Thus, the majority of the effects of Ang II are mediated by AT1a receptors (Gasparo et al., 2000; Kobori et al., 2007b; Forrester et al., 2018; Li et al., 2018b, 2021). Indeed, over 95% of the renal AT1 receptors belong to the AT1a subtype, whereas AT1b receptors account for only about 5% (Chen et al., 1997). The anatomic and cellular localization of AT1 (AT1a) receptors in the kidney has been well characterized using quantitative autoradiography with radiolabeled Ang II (Zhuo et al., 1992), in situ hybridization histochemistry (Aguilera et al., 1994), and immunohistochemistry (Harrison-Bernard et al., 1997). However, radioreceptor binding assays and autoradiography remains the gold-standard approach due to its highly specific ligand and receptor interactions. Indeed, we and others have used this approach to localize AT1 (AT1a) receptors in the kidneys of rat, mouse, rabbit, monkey, or humans with a striking pattern of anatomic and cellular distribution (Fig. 5) (Gibson et al., 1991; Grone et al., 1992; Sechi et al., 1992; Zhuo et al., 1992, 1993, 1996). Autoradiographs show that in the cortex, a very high density of AT1 receptor binding occurs in the glomerulus, where it dominates in mesangial cells and less in endothelial cells, epithelial cells, and podocytes. A moderate level of AT1 receptor binding is localized over the intervening outer cortex, primarily corresponding to the proximal convoluted tubules (Grone et al., 1992; Sechi et al., 1992; Zhuo et al., 1992, 1993). AT1 receptor binding is low to undetectable in the outer stripe of the outer medulla, where the loop of Henle is primarily located. By comparison, very high density AT1 receptor binding is localized in the longitudinal bands traversing the inner stripe of the outer medulla associated with the vasa recta bundles. In the interbundle area of the inner stripe of the outer medulla, there is a moderate density of AT1 receptor binding primarily associated with type 1 renomedullary interstitial cells (RMICs). Interestingly, the entire inner medulla (IM) of the kidney, especially toward the tip of the IM, expresses a very low level of AT1 receptor binding (Fig. 5). The inner medullary CDs are primarily located in this region. Consistent with the well recognized hemodynamic effect, Ang II receptor binding is also seen overlying the media of intrarenal blood vessels but with a much lower density than the binding associated with glomeruli, proximal convoluted tubules, or the vasa recta bundles.
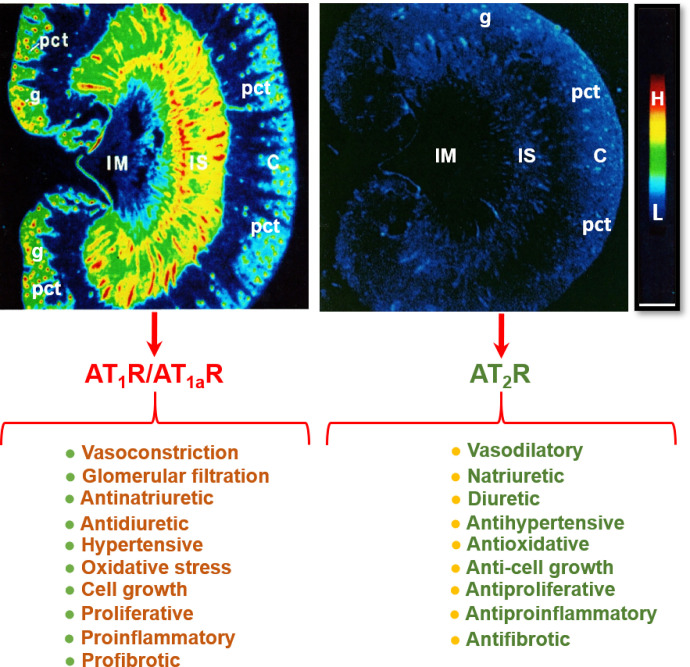
Localization of angiotensin II type 1 receptor (AT1R, largely representing its subtype a, AT1aR) and angiotensin II type 2 receptor (AT2R) in the rat kidney using quantitative in vitro autoradiography and opposing actions of AT1R/AT1aR and AT2R in the kidney. Panel A shows the anatomic localization of AT1R/AT1aR with high levels in the glomerulus (g) and the inner stripe of the outer medulla corresponding to vasa recta bundles, and moderate levels in the proximal convoluted tubules (pct) in the cortex (pct) and renomedullary interstitial cells (RMICs) in the inner stripe of the outer medulla between vasa recta bundles. The inner medulla (IM) expresses a very low level of AT1R/AT1aR. Panel B shows the anatomic localization of AT2R, with low levels in the outer cortex, corresponding to the glomeruli and the proximal tubules, and the inner stripe of the outer medulla, corresponding to vasa recta bundles and RMICs. Again, the IM expresses a very low level of AT2R. Red represents high level (H), whereas dark blue represents background levels (L). Modified from Zhuo et al. (1992, 1994).
In situ hybridization histochemistry also offers a high specificity to localize AT1 (AT1a) mRNA expression in the kidney at the light microscopic level. Due to the sensitivity of the approach, AT1 (AT1a) mRNA expression is widely seen throughout the kidney, including the blood vessels, glomerulus, proximal tubules, loop of Henle, distal tubules, and collecting ducts (Aguilera et al., 1994; Gasc et al., 1994; Healy et al., 1995). By contrast, the approach of localizing AT1 (AT1a) receptors in the kidney using immunohistochemistry with AT1 receptor antibodies remains highly controversial. The key issue with using immunohistochemistry to localize AT1 receptor proteins in the kidney or other tissues is that all commercially available AT1 receptor antibodies are not specific to AT1 receptors (Herrera et al., 2013), thus leading to false positive localization of AT1 receptors. Immunohistochemistry is therefore not advised to be used for AT1 receptor localization.
B. AT2 Receptor
The AT2 receptor is a seven-transmembrane G protein–coupled receptor. The AT2 receptor is highly expressed during fetal life (Grady et al., 1991), suggesting that it may play an important role during development. Although AT2 receptor expression generally declines after birth, persistent AT2 receptor expression can be detected in several adult tissues, including the adrenal glands, kidneys, uterus, ovaries, vasculature, heart, and brain (Matsubara et al., 1998; Sampson et al., 2012). In the adult kidney, the AT2 receptor is widely expressed (albeit at low levels) in the vasculature (Zhuo et al., 1996; Matsubara et al., 1998), glomeruli, and tubular segments (Miyata et al., 1999; Bosnyak et al., 2010). Stimulation of the AT2 receptor generally opposes the classic effects of AT1 receptor stimulation by inducing vasodilation, natriuresis, and antifibrotic and anti-inflammatory effects (Fig. 5). The vasodilatory effects of the AT2 receptor are mediated via an increase in the production of nitric oxide (NO) and cGMP, which is achieved either by increasing bradykinin production with a subsequent effect mediated through bradykinin type 2 receptors or directly via activation of NO production independent of bradykinin (Padia and Carey, 2013). However, there are reports of AT2 receptor–mediated vasoconstriction as well as no effect of AT2 receptor stimulation on vascular tone, as reviewed previously (Verdonk et al., 2012a). Similarly, there are numerous studies that have reported no effect of AT2 receptor stimulation or blockade on renal function (Uhlenius et al., 2002; Chappellaz and Smith, 2007; Welch et al., 2007). The discrepancy between these findings may be related, at least in part, to the specificity of the tools used to interrogate the function of the AT2 receptor and the relative AT2:AT1 receptor ratio, which is integral in determining the effects of RAS stimulation on vascular tone and kidney function. For example, the nonpeptide AT2 receptor agonist compound 21 (C21), which is 4000-fold more selective for the AT2 receptor than the AT1 receptor, is not specific for the AT2 receptor at higher doses (i.e., within the micromolar range) (Verdonk et al., 2012b). As discussed below, accumulating evidence suggests that renal AT2 receptor expression and function is greater in females than in males (Fig. 6). Of note, recent studies utilizing RNA sequencing to profile the entire length of the nephron (performed almost exclusively using tissues obtained from adult male rodents) have demonstrated that expression of the AT2 receptor is low to negligible (Chen et al., 2021). This may explain why the effects of AT2 receptor stimulation are often only unmasked in the presence of an ARB (Padia and Carey, 2013) and that recent studies investigating the natriuretic effects of the AT2 receptor have been performed largely in female rodents.
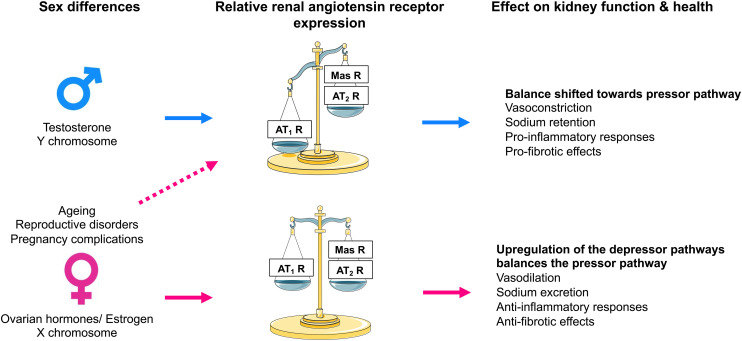
Effect of sex on renal angiotensin receptor expression and kidney function and health. Expression of the angiotensin II type 2 receptor (AT2R) and Mas receptor are differentially modulated by sex chromosomes and sex hormones and are influenced by female-specific conditions and diseases. Consequently, in males, the relative renal angiotensin receptor balance is skewed toward the angiotensin II type 1 receptor (AT1R), which enhances vasoconstriction, sodium reabsorption and proinflammatory and profibrotic effects within the kidney. Conversely, adult females have greater expression of the AT2 and Mas receptors than males, which counterbalances the effect of the AT1 receptor and enhances vasodilation, natriuresis and anti-inflammatory and antifibrotic effects within the kidney. In females, various factors such as age, menopause, and complications of pregnancy can lead to a reduction in the renal expression of AT2 and Mas receptors, leading to an increase in blood pressure and sodium retention and adverse effects on kidney health. MasR, Mas receptor.
Targeted disruption of the mouse Agtr2 gene has demonstrated that AT2 receptor deficiency results in a rightward shift of the pressure-natriuresis relationship (Gross et al., 2000), increased salt-sensitivity (Siragy et al., 1999), and enhanced sensitivity to Ang II infusion, which is associated with reduced urinary sodium excretion (Siragy et al., 1999) and bradykinin and cGMP levels (Gross et al., 2000). In rats, both systemic and intrarenal infusion of C21 has been demonstrated to induce natriuresis, and this effect was completely abolished by concomitant intrarenal infusion of the AT2 receptor antagonist PD123319 (Hilliard et al., 2012; Kemp et al., 2016). Since AT2 receptor activation increases renal blood flow but not GFR (Hilliard et al., 2012, 2014; Kemp et al., 2014, 2016), AT2 receptor–mediated natriuresis must be due to changes in tubular function rather than renal hemodynamics. Within the proximal tubule, AT2 receptor–mediated natriuresis is achieved by translocation of AT2 receptors to the apical plasma membrane of proximal tubular cells (Kemp et al., 2014, 2016; Krause et al., 2020), inhibition of HCO3− reabsorption (Haithcock et al., 1999), and the translocation, internalization, and inactivation of NHE3 in the apical membrane and Na+/K+-ATPase in the basolateral membrane (Kemp et al., 2014). The latter occurs via the NO/cGMP pathway. The AT2 receptor also decreases AT1 receptor expression and function via the NO/cGMP/specificity protein 1 serine phosphorylation pathway (Yang et al., 2012a). Additionally, activation of the AT2 receptor reduces renal fibrosis either directly or indirectly via its anti-inflammatory effects and stimulation of the NO/cGMP pathway. In the 2-kidney, 1-clip hypertension model (Matavelli et al., 2011) and stroke-prone SHRs (Rehman et al., 2012), AT2 receptor agonism with C21 reduced renal inflammation and fibrosis, which was associated with improved production of NO and cGMP.
Accumulating evidence suggests that Ang III is the endogenous intrarenal ligand for the AT2 receptor. Studies in normotensive rat kidneys have demonstrated that Ang III, but not Ang II, induced AT2 receptor–mediated natriuresis (Padia et al., 2007) and that this effect was mediated via activation of proximal tubule AT2 receptors via a cGMP-dependent pathway (Kemp et al., 2012). Similar findings have been reported recently in response to a novel β-amino acid substituted Ang III, β-Pro7-Ang III, which has high specificity for the AT2 receptor (Krause et al., 2020). Further, the Ang III–induced AT2 receptor–mediated natriuretic effect was augmented by the blockade of aminopeptidase N, an enzyme metabolizing Ang III to Ang IV, thereby increasing Ang III levels (Padia et al., 2007; Kemp et al., 2012). Interestingly, Ang III–induced AT2 receptor–mediated natriuresis is not observed in SHRs (Padia et al., 2009), indicating that a deficit in AT2 receptor–mediated natriuresis contributes to the development of hypertension in this model.
The location of the Agtr2 gene on the X chromosome suggests that the AT2 receptor may have sexually dimorphic actions. Using the four-core genotype model, where the Sry gene is translocated to chromosome 3, making it possible to differentiate between effects that are sex hormone and/or sex chromosome complement dependent (Pessôa et al., 2015), it has been demonstrated that the X chromosome increases renal AT2 receptor expression (Dadam et al., 2017). Furthermore, numerous animal studies have demonstrated that renal AT2 receptor expression is greater in females than in males (Hilliard et al., 2014; Mirabito et al., 2014) and that this effect is linked to either estrogen, ovarian hormones, or reproductive status (Mirabito et al., 2014; Barsha et al., 2021). Thus, the AT2 receptor may contribute to the relative cardiorenal protection observed in adult females compared with age-matched males and reproductively senescent (e.g., postmenopausal) females.
Studies in rodents provide strong evidence of an enhanced functional role for renal AT2 receptors in females (Fig. 6). For example, genetic AT2 receptor deficiency resulted in a rightward shift of the chronic pressure-natriuresis relationship in adult female mice compared with their wild-type and age-matched male counterparts (Mirabito et al., 2014). In anesthetized rats, acute pharmacological stimulation of the AT2 receptor with graded doses of C21 (Hilliard et al., 2012) and the highest dose of β-Pro7-Ang III tested (Krause et al., 2020) produced a greater renal vasodilator response in normotensive females than in males. Moreover, in female rats, the vasodilatory and natriuretic effects induced by C21 were of a slightly greater magnitude in normotensive rats than in SHRs. These responses to C21 treatment were completely absent in male SHRs (Hilliard et al., 2014). In mice, Ang II–induced contraction in interlobar arteries was significantly attenuated in females compared with males via an AT2 receptor–mediated NO-dependent pathway (Viegas et al., 2012). Hilliard et al. (2011) have shown that at low renal perfusion pressures in female rats, the AT2 receptor maintains autoregulation of renal blood flow and GFR. Furthermore, the sensitivity of the tubuloglomerular feedback mechanism to Ang II is reduced by the presence of the AT2 receptor in female but not male mice (Brown et al., 2012). There is evidence to suggest that the enhanced functional role of renal AT2 receptors in females diminishes with age. In association with a reduction in renal AT2 receptor expression, the chronic pressure-natriuresis relationship is shifted rightward (Mirabito et al., 2014) and the hypertensive response to Ang II is enhanced (Barsha et al., 2021) in aged reproductively senescent females compared with their adult counterparts. In these studies, genetic or pharmacological AT2 receptor deficiency did not alter the response in the aged females. Excitingly, in aged females, estrogen replacement reduced the pressor response to Ang II to that observed in adult females and this effect was associated with an upregulation in renal AT2 receptor expression (Barsha et al., 2021), suggesting that estrogen replacement may restore the effects of the AT2 receptor on kidney function in aged females.
Although sex differences in AT2 receptor expression and function have not been examined in humans, there is evidence to suggest a greater functional role for the AT2 receptor in women than in men. For example, in healthy adults the renal pressor response to Ang II was blunted in women compared with men (Miller et al., 2006), and in response to treatment with an ARB, Ang II sensitivity is decreased to a greater extent in women than in men (Hudson et al., 2007). Finally, renal APA levels are greater in women than in men (Martínez et al., 1998), suggesting higher levels of Ang III and an enhanced AT2 receptor–mediated natriuretic response in women.
Of interest, expression of the AT2 receptor can be upregulated during pathologic settings such as myocardial infarction and kidney damage (Oishi et al., 2003; Vázquez et al., 2005), which has led to the notion that AT2 receptor agonism may be a novel therapeutic option for cardiovascular disease. Yet, preclinical studies indicate that AT2 receptor agonists are not suitable as a monotherapy for cardiovascular disease and that at best, AT2 receptor agonists may be beneficial in combination with existing RAS inhibitors (Danyel et al., 2013). Currently, the clinical focus of AT2 receptor agonists (in development by Vicore Pharma) is severe lung disorders, with C21 receiving orphan drug status for idiopathic pulmonary fibrosis by the US Food and Drug Administration and the European Medicines Agency and a post hoc analysis of a recent phase 2 clinical trial, suggesting that 7-day treatment with C21 in hospitalized patients with COVID-19 reduced the requirement for oxygen at day 14 (Tornling et al., 2021).
C. Mas Receptor
The Mas receptor was first described in 1986 as Mas oncogene (Young et al., 1986), and it was not until 2003 that the Mas receptor was identified as a G protein–coupled receptor with high affinity for Ang-(1-7) (Santos et al., 2003). Within the kidney, the Mas receptor is expressed in afferent arterioles and the apical surface of tubular epithelium (Alenina et al., 2008). Upon stimulation with Ang-(1-7), the Mas receptor undergoes endocytosis (Gironacci et al., 2011) and is then slowly recycled back to the plasma membrane (Cerniello et al., 2017). Similar to the AT2 receptor, stimulation of the Mas receptor generally opposes the effects of the AT1 receptor stimulation by inducing vasodilation, natriuresis, and antifibrotic and anti-inflammatory effects. In the vasculature, Ang-(1-7)/Mas receptor stimulation increases NO release by inducing protein kinase B phosphorylation and the activation of endothelial NO synthase and inhibits endothelial Ang II–induced ROS production, leading to improved endothelial function (Sampaio et al., 2007a,b). Further, Ang-(1-7) has been demonstrated to potentiate bradykinin-induced vasodilation in various models, including porcine coronary arteries, canine coronary rings, and bovine aortic endothelial cells, an effect that is diminished by nitric oxide synthase inhibition and the bradykinin type 2 receptor antagonist HOE 140 (Li et al., 1997; Heitsch et al., 2001; Tom et al., 2001). The Mas receptor also hetero-oligomerizes with the AT1 receptor to act as a physiologic antagonist of the AT1 receptor (Kostenis et al., 2005). Consequently, Mas receptor–deficient mice have impaired endothelial function and higher blood pressure than their wild-type counterparts (Xu et al., 2008). Within the kidney, Ang-(1-7) alters sodium and HCO3− reabsorption and stimulates the release of NO, PGE2, and prostaglandin I2 (PGI2) and augments the vasodilator effects of bradykinin (Chappell, 2012). Here it should be noted that the renal salt-handling response to Ang-(1-7)/Mas receptor stimulation (i.e., whether it will induce natriuresis or antinatriuresis) depends on a number of factors, including water and electrolyte balance, species, sex, pregnancy, nephron segment, and disease. Nevertheless, the ACE2/Ang-(1-7)/Mas receptor axis and the AT2 receptor are often collectively referred to as the protective RAS pathways.
The majority of studies have demonstrated that Ang-(1-7) exerts natriuretic and diuretic effects via activation of the Mas receptor (DelliPizzi et al., 1994; Santos et al., 1996). Within the proximal tubule, Mas receptors alter sodium, HCO3−, and fluid reabsorption via NHE3 (Castelo-Branco et al., 2013). Ang-(1-7) also inhibits sodium transport in the thick ascending loop of Henle via Mas receptor–dependent increases in NO (Dibo et al., 2019). Accumulating evidence also indicates that AT1 and AT2 receptors contribute to the natriuretic and diuretic effects of Ang-(1-7) (Patel et al., 2017), although the exact mechanisms remain unclear. For example, studies suggest that the Mas receptor can interact with the AT1 receptor and prevent its activation either by acting directly at the AT1 receptor (Galandrin et al., 2016) or indirectly by heterodimerizing with the AT1 receptor to act as a physiologic antagonist (Kostenis et al., 2005). Conversely, in certain settings, including in normotensive and hypertensive rats (Simões e Silva et al., 1998), after an acute water load (Santos et al., 1996), and in virgin females (Joyner et al., 2008), Ang-(1-7) can induce antidiuresis. This effect appears to be independent of vasopressin (Santos and Baracho, 1992) and is instead mediated via effects of Ang-(1-7) on proximal tubules (Garcia and Garvin, 1994) and inner medullary collecting ducts (Santos et al., 1996). In addition to the tubular effects of Ang-(1-7)/Mas receptor stimulation, studies in normotensive and hypertensive rats have demonstrated that renal blood flow is also modulated by Ang-(1-7) and that this effect is abolished by blockade of the Mas receptor or inhibition of prostaglandin release and NO, which are thought to be downstream of Mas receptor activation (Sampaio et al., 2007b).
Compared with wild-type mice, Mas receptor KO mice have decreased urine volume and natriuresis, similar free water clearance, and increased inulin clearance and microalbuminuria concomitant with a reduced renal blood flow, suggesting hyperfiltration (Pinheiro et al., 2009). Histologic examination of the kidneys of these mice indicates that Mas receptor deficiency impairs the glomerular filtration barrier, as evidenced by a reduction in the glomerular tuft diameter, and promotes a profibrotic phenotype with increased expression of fibronectin and collagens III and IV (Pinheiro et al., 2009). Importantly, these changes were associated with increased renal mRNA expression of the AT1 receptor and TGFβ1 (Pinheiro et al., 2009). Similarly, in models of obstructive and DKD, it has been demonstrated that Ang-(1-7) reduced TGFβ1 expression and signaling through TGFβ1/Smad complex and that these effects were reversed by Mas receptor blockade (Liu et al., 2012). Ang-(1-7)/Mas receptor also modulates MAPKs, which may contribute to its antioxidant, anti-inflammatory, and antifibrotic effects. For example, in the presence of Ang II or high glucose in proximal tubule cells, the binding of Ang-(1-7) to the Mas receptor inhibits phosphorylation of MAPKs, including p38, ERK1/2, and c-Jun N-terminal kinase (Su et al., 2006; Gava et al., 2009). Conversely, in dual ACE2 and Mas receptor–deficient mice, Ang II–induced hypertension resulted in severe hypertensive nephropathy, which was associated with decreased creatinine clearance and increased renal fibrosis, inflammation, and AT1 receptor-ERK1/2-Smad3 and NF-κB signaling (Ni et al., 2020). Collectively, these findings support a key role for the ACE2/Ang-(1-7)/Mas receptor axis in the maintenance of kidney health. Similar to the AT2 receptor, evidence suggests a sex-specific role for the ACE2/Ang-(1-7)/Mas receptor axis. Renal mRNA expression of ACE2 and Mas receptor and renal cortical Ang-(1-7) levels are greater in female versus male rodents (Sullivan et al., 2010; Sampson et al., 2012; Mirabito et al., 2014; Zimmerman et al., 2015). Moreover, in both normotensive and hypertensive rats, it has been reported that exogenous Ang II infusion induces a greater increase in renal cortical Ang-(1-7) levels in females than in males (Sullivan et al., 2010; Zimmerman et al., 2015). Interestingly, Mas receptor expression was increased in the renal cortex after Ang II infusion in females only (Sullivan et al., 2010), suggesting that Ang-(1-7) elicits its effects via the AT2 receptor in adult males. Consistent with this notion, it has been demonstrated that Ang-(1-7) elicits a vasodepressor response in adult males, which is blocked by coadministration of the AT2 receptor antagonist PD123319 (Bosnyak et al., 2012). Further, in the presence of Mas receptor blockade, renal blood flow decreased significantly in anesthetized female but not male normotensive rats (Safari et al., 2012).
In contrast to the studies that suggest a protective role for the ACE2/Ang-(1-7)/Mas receptor axis, accumulating evidence suggests that downregulation of this pathway may be beneficial in disease settings. For example, genetic and pharmacological Mas receptor deficiency has been reported to prevent high-fat diet–induced kidney injury (Kong et al., 2021), abolish salt-sensitive hypertension (Heringer-Walther et al., 2012), reduce kidney damage in models of kidney injury (e.g., unilateral ureteral obstruction and ischemia/reperfusion injury) (Esteban et al., 2009), and inhibit NF-κB activation and thus the upregulation of proinflammatory cytokines (Esteban et al., 2009). Clearly, more studies are needed to establish the contribution of the ACE2/Ang-(1-7)/Mas receptor axis in kidney health and disease, also in view of the fact that recent studies do not support that Ang-(1-7) is the endogenous agonist of the Mas receptor (Gaidarov et al., 2018). Moreover, renal Ang-(1-7) levels, if detectable at all, are usually far below those of Ang II (Campbell et al., 1995a; Roksnoer et al., 2015; Bovée et al., 2021; Uijl et al., 2021). This raises the question of whether Ang-(1-7)-Mas receptor interaction truly occurs under physiologic conditions.
D. Localization and Role of Intracellular Angiotensin Receptors in the Kidney
The renal effects of Ang II mediated by cell surface receptors have been extensively studied (Kobori et al., 2007b). However, whether Ang II receptors are present intracellularly, and if so, where they are localized in the cell, and whether intracellular Ang II receptors mediate any biologic or physiologic effects of intracellular Ang II remains poorly understood. The concept of an intracrine or intracellular Ang II system is not new. Initially, two possibilities emerged to explain the ‘local’ effects of Ang II. First, that Ang II is generated intracellularly and then released into the extracellular fluid compartment to induce effects via cell membrane receptors in an autocrine or paracrine manner. Second, that Ang II is generated extracellularly, on the cell surface by membrane-bound ACE, and then acts on neighboring membrane receptors. Of course, after intracellular expression, AT receptors will appear on the cell membrane, whereas after Ang II binding, cell membrane AT1 receptors are expected to internalize (potentially resulting in desensitization), with internalized Ang II subsequently being degraded in the lysosomal pathway. As discussed earlier, the second possibility now is the most likely, given that renal intracellular Ang II disappeared in animals that lack all AT receptors (van Kats et al., 2001b; van Esch et al., 2010b), whereas conceptually it is hard to envision a combination of either internalization or intracellular expression of renin, AGT, and ACE, allowing them to perform their reaction cascade in the same intracellular compartment.
However, there is accumulating evidence supporting the presence of intracellular Ang II that plays important roles in diverse biologic and physiologic responses (Cook et al., 2001; Zhuo et al., 2006; Li and Zhuo, 2008; Li et al., 2011, 2020). This Ang II may simply represent internalized Ang II of extracellular origin. In the rat liver, early studies have reported that Ang II receptor binding sites are present in isolated nuclei in which Ang II directly induced effects, including AGT transcription (Eggena et al., 1996). In the heart, administration via intracellular dialysis of renin, AGT, or Ang II directly into hamster cardiomyocytes was demonstrated to alter intercellular communications (De Mello, 1998), whereas in VSMCs, microinjection of Ang II directly into the cells elicited intracellular and nuclear calcium responses (Haller et al., 1996). As these intracellular responses to intracellular Ang II administration were blocked by concurrent intracellular administration of ARBs, these early studies support the proof-of-concept hypothesis that intracellular AT1 receptors mediated these effects. More recent studies on intracellular Ang II and Ang II receptors involved the development and application of an intracellular cyan fluorescent Ang II fusion protein, ECFP/Ang II (Cook et al., 2001; Li et al., 2011, 2020). This Ang II fusion protein is constructed to be expressed intracellularly without being released into the extracellular fluid compartment, and it only acts on intracellular AT receptors to induce intracellular effects.
In the kidney, intracellular AT1 (AT1a) and AT2 receptors have been reported in freshly isolated endosomes (Zhuo et al., 2002), endoplasmic reticulum (Ferrão et al., 2017), mitochondria (Li et al., 2020), and nuclei from the renal cortex (Li and Zhuo, 2008). Most of these intracellular AT receptors in the renal cortex are associated primarily with the proximal tubules of the kidney and are likely derived from both intracellular expression and Ang II–induced internalization and subsequently trafficking to endoplasmic reticulum, mitochondria, and nucleus. Whether intracellular AT receptors are localized in other renal cells has not been studied. In contrast to the mainstream views, not all internalized Ang II and AT1 (AT1a) receptors are sorted into the lysosomal degradation pathways, especially in a chronic and high Ang II environment in the in vivo setting (van Kats et al., 1997, 2001b; Zhuo et al., 2002; Li et al., 2007, 2009; Wilson et al., 2016). Some internalized Ang II and AT1 (AT1a) receptors bypass the lysosomal degradation pathways and are transported to other intracellular organelles. Thus, internalized Ang II may serve as an important source of intracellular Ang II (Fig. 7), which may stimulate its cytoplasmic and nuclear AT1 or AT2 receptors to induce important biologic and physiologic effects.
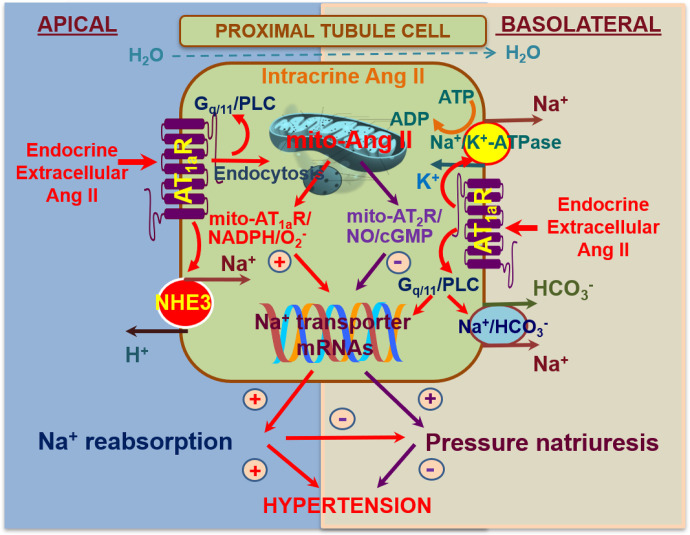
Scheme showing that extracellular (endocrine and paracrine) angiotensin (Ang) II is taken up via Ang II type 1a receptor (AT1aR)-mediated internalization and then results in Gq/11/phospholipase C (PLC) signaling. Under physiologic conditions (low Ang II), internalized Ang II is sorted to the lysosomal pathway for degradation, whereas AT1a receptors recycle back to the membrane. Alternatively, particular at sustained high extracellular Ang II levels, the Ang II–AT1aR complex may bypass the lysosomal degradation pathway, allowing its transport to mitochondria and nucleus, where Ang II activates AT1aR and/or Ang II type 2 receptor (AT2R) to alter mitochondrial oxidative and glycolysis stress responses. This may in turn alter the expression or activity of Na+/H+ exchanger 3 (NHE3) on the apical membranes, or Na+/K+-ATPase and the Na+/HCO3− cotransporter on the basolateral membranes in the proximal tubules. Thus, activation of the mitochondrial Ang II/AT1aR/O2− signaling will stimulate proximal tubule sodium reabsorption, impair the pressure-natriuresis response, and elevate blood pressure. Conversely, activation of the mitochondrial Ang II/AT2R/NO/cGMP signaling by overexpressing AT2R in the mitochondria will likely inhibit proximal tubule sodium reabsorption, augment the pressure-natriuresis response, and lower blood pressure. Modified from Li et al. (2020, 2021).
This hypothesis has been tested in both cultured proximal tubule cells and the proximal tubules of the kidney, and the data support an important biologic and physiologic role of intracellular Ang II via acting on intracellular, mitochondrial, and nuclear AT1 (AT1a) and AT2 receptors (Zhuo et al., 2006; Li and Zhuo, 2008; 2013; Li et al., 2011, 2020, 2021). Early in vitro studies demonstrated that microinjection of Ang II directly into cultured rabbit proximal tubule cells significantly increased intracellular Ca2+ responses, even during blockade of cell surface AT1 receptors with losartan (Zhuo et al., 2006). Since concurrent microinjection of losartan prevented the Ca2+ response, it is likely induced via activation of intracellular AT1 receptors (Haller et al., 1996; Zhuo et al., 2006). In freshly isolated rat renal cortical nuclei, incubation with Ang II significantly increased transcriptional responses on TGFβ1, monocyte chemoattractant protein-1, and NHE3 mRNA expression (Li and Zhuo, 2008). Since freshly isolated nuclei were completely devoid of endosomes, lysosomes, endoplasmic reticulum, mitochondria, and cell membranes, these Ang II–induced transcriptional responses must have been due to direct actions of Ang II via activation of nuclear AT receptors (Li and Zhuo, 2008). These in vitro effects of intracellular Ang II were further supported by in vivo animal studies in which an intracellular Ang II fusion protein was expressed selectively in the proximal tubules of the kidney (Li et al., 2011, 2020, 2021). Adenovirus-mediated overexpression of the intracellular Ang II fusion protein selectively in the mitochondria of the proximal tubule cells induced two key mitochondrial functional responses: oxygen consumption rate and extracellular acidification rate (Li et al., 2020). Finally, overexpression of this Ang II fusion protein in the mitochondria of the proximal tubules in mice increased blood pressure, and this effect was attenuated in mutant mouse models with proximal tubule–selective deletion of either AT1a receptors or NHE3 (Li et al., 2020, 2021). This suggests that intracellular Ang II in the mitochondria of the proximal tubules may play an important role in the physiologic regulation of proximal tubule sodium reabsorption and blood pressure homeostasis.
IV. Intrarenal RAS and Disease
A. Hypertension
It is widely believed that the intrarenal RAS plays a central role in the development and maintenance of hypertension. Here the acute effects of Ang II on salt and water retention are obvious players, particularly when combined with the long-term effects of Ang II on growth, remodeling, and inflammation. The activation of the renal RAS does not necessarily run in parallel with that of the systemic RAS (Navar et al., 2002; Kobori et al., 2007b). Initially this was attributed to upregulated local expression of AGT in the proximal tubule (e.g., in response to systemic Ang II infusion). Although kidney-specific AGT overexpression in transgenic mice indeed resulted in hypertension (Davisson et al., 1999), we now know, as discussed above in section II.C, that the role of locally synthesized AGT in the kidney is marginal, if not absent. A more likely scenario is that high blood pressure (e.g., after infusing Ang II) affects glomerular filtration, thus resulting in the accumulation of filtered hepatic AGT into the kidney and allowing increased Ang II generation (Koizumi et al., 2019). Since hypertension will normally suppress renin release (Danser et al., 1998), it is not unlikely that low systemic RAS activity indeed occurs in combination with high renal RAS activity (due to enhanced AGT uptake).
Given that both circulating renin and renin at renal tissue sites derive from JG cells, it is difficult to conceive a situation where local renin release is upregulated while systemic release is down. It is for this reason that CD (pro)renin production seemed an attractive concept. As discussed in section II.B, chronic Ang II infusion would then upregulate CD (pro)renin (Prieto-Carrasquero et al., 2004) and simultaneously suppress JG renin synthesis, thus again resulting in the combination of low systemic renin and high renal/CD RAS activity, with the latter potentially involving the (P)RR. However, given the absence of renin lineage cells at the level of the CD (Tang et al., 2019), local (pro)renin synthesis at this location seems impossible. An alternative explanation would be that CD (pro)renin represents enhanced filtered circulating (pro)renin, particularly since Ang II not only impairs the glomerular filtration barrier but also downregulates megalin, thereby preventing (pro)renin reabsorption in the proximal tubule (see section II.B).
Renal ACE is another contributor to hypertension, and various hypertension models support its upregulation under hypertensive conditions (Gonzalez-Villalobos et al., 2013; Giani et al., 2014). Here an obvious question is whether this is the cause or consequence of hypertension. Mice expressing ACE exclusively in the kidney tubules but not in other tissues displayed hypertension when infused with Ang I (Gonzalez-Villalobos et al., 2011). Yet, mice devoid of renal ACE were resistant to hypertension induced by Ang II infusion or the NO synthase inhibitor NG-nitro-L-arginine methyl ester (L-NAME) (Gonzalez-Villalobos et al., 2013; Giani et al., 2014). The authors argued that both Ang II infusion and L-NAME treatment upregulated renal ACE 1.5- to 2-fold, thus enhancing local Ang II generation and sodium transporter activation and subsequently leading to hypertension via a reduction in natriuresis. The same authors showed that mice specifically expressing ACE in renal tubular epithelium developed salt-sensitive hypertension in response to L-NAME, whereas mice selectively lacking renal tubular epithelial ACE did not. Thus, it is particularly Ang II generated by tubular epithelial ACE that contributes to salt-sensitive hypertension (Giani et al., 2017). A caveat of these studies is that renal ACE in the mice that were supposed to lack renal ACE was not zero but rather in the order of 10%–15% of wild-type levels. This resembles what can be achieved during ACE inhibition (see section V.A) and raises the question of what the renin levels were in these models. Normally, such a reduction of ACE activity can be easily matched by 5- to 10-fold rise in renin. Thus, before definitely invoking a modest rise in renal ACE as the underlying cause of the blood pressure rise, future studies should rule out that it is not simply due to changes in renin.
Crowley et al. (2005, 2006) used a kidney crosstransplantation strategy to generate mice expressing AT1 receptors either only within or only outside the kidney, thus demonstrating that activation of renal AT1 receptors induced Ang II–dependent hypertension whereas exclusive extrarenal AT1 receptor stimulation was insufficient to cause hypertension. These results support a pivotal role of renal AT1 receptors in blood pressure regulation. Moreover, proximal tubule–specific AT1 receptor ablation lowered blood pressure and prevented Ang II–induced hypertension by reducing sodium and fluid reabsorption (Gurley et al., 2011; Li et al., 2021).
Taken together, changes in renal AT1 receptor density combined with alterations in local ACE and the reabsorption of filtered (pro)renin and AGT allow an upregulation of Ang II–mediated effects in the kidney, even in the absence of changes in the circulating RAS or when circulating RAS activity is low. These local effects of Ang II may contribute to both the development and maintenance of hypertension, and this explains why RAS blockers are also effective in patients with low to normal circulating RAS activity.
B. Chronic Kidney Disease
The renoprotective effects of RAS inhibitors beyond blood pressure control have been recognized since the 1990s. ARBs and ACEIs have been the first line of therapy for hypertensive chronic kidney disease (CKD) ever since (Jafar et al., 2001), especially in patients with albuminuria or DKD (Palmer et al., 2015). A prevailing hypothesis is that the initial kidney injury activates the intrarenal RAS causing local Ang II production, thereby driving CKD progression. This is not surprising since Ang II not only causes vasoconstriction and increases glomerular pressure and sodium retention but, in addition to these glomerular and tubular effects, also induces inflammation, fibrosis, and glomerular sclerosis, thereby leading to further nephron loss (Rüster and Wolf, 2006). To address the role of the intrarenal RAS, most studies have relied on measuring RAS components in kidney tissue or urine. Here it is difficult to distinguish the circulating and renal RAS, except in the case of ACE and AT receptors.
An increase in renal RAS components has been observed in several animal models of CKD. For example, in models of kidney mass reduction, tubular renin and Ang II are increased and early RAS inhibition prevents a further GFR decline (Gilbert et al., 1999). Animals who are made diabetic with STZ generally show an increase in renal Ang II and urinary AGT prior to a decrease in GFR and the development of albuminuria (Singh et al., 2005; Kamiyama et al., 2012). In other diabetic animal models, including Zucker rats (a genetic obesity animal model caused by mutation in the leptin receptor), Otsuka Long-Evans Tokushima Fatty rats, and db/db mice, kidney Ang II and AGT are increased both before and after the development of kidney damage (Nagai et al., 2005; Miyata et al., 2008), although not all investigators agree (Leehey et al., 2008). Finally, in rodent models of glomerular disease, including IgA nephropathy and antithymocyte serum nephritis, an increase in kidney RAS components compared with healthy controls was observed (Ohashi et al., 2008; Huang et al., 2012).
Several groups have reported that RAS component expression in human kidney biopsies correlates with the presence and severity of the underlying kidney disease (Lai et al., 1998; Del Prete et al., 2003; Mezzano et al., 2003; Konoshita et al., 2006; Kobori et al., 2007a; Takamatsu et al., 2008; Ohashi et al., 2017; Zhang et al., 2019). In patients with mixed etiology kidney disease, RAS component mRNA levels were upregulated in glomeruli and tubules compared with controls (Lai et al., 1998). Renal tissue AGT and AT1 receptor density associated with the degree of fibrosis in patients with kidney failure (Ohashi et al., 2017). In kidney biopsies taken from patients with DKD and IgA nephropathy, higher RAS abundance has been consistently observed. Patients with DKD show increased RAS abundance in tubular and interstitial cells compared with healthy controls (Mezzano et al., 2003) and hence respond less strongly to RAS blockade (Price et al., 1999; Lansang et al., 2001). Kidney biopsies from patients with DKD also show increased ACE mRNA compared with patients with non-DKD CKD (Konoshita et al., 2006). In patients with IgA nephropathy, an overexpression in RAS genes has been observed (Del Prete et al., 2003). IgA nephropathy increased AGT and Ang II immunoreactivity along the tubule, and AGT was present in glomeruli (Kobori et al., 2007a). Greater AGT and Ang II immunoreactivity was also observed in glomeruli from pediatric patients with IgA nephropathy (Takamatsu et al., 2008). Expression of the AT1 receptor in patients with IgA nephropathy is related to disease severity and reduced by RAS inhibition (Zhang et al., 2019).
Although generally the circulating RAS is up in CKD, it is not in patients with diabetes, whose plasma renin levels are low (Price et al., 1999). This has been known for decades, and simultaneously the remarkable observation was made that circulating prorenin in patients with diabetes is elevated (Luetscher et al., 1985). Since prorenin levels in patients with diabetes correlated with microvascular complications, including nephropathy and retinopathy, initial studies focused on the eye as a potential source of this prorenin. Although this led to the discovery that prorenin is indeed made in the eye, its relatively low ocular levels, combined with the low ocular flow, made the release of significant quantities of prorenin from the eye into the blood stream unlikely (Danser et al., 1989). Next, the concept of the CD as the source of this prorenin, particularly in combination with the (P)RR as a prorenin activator, caused excitement (Kang et al., 2008). However, as discussed in section II.B, this concept is now also being abandoned, leaving the possibility that the elevated prorenin levels in diabetes originate in the JG cells after all. Whether this prorenin underlies the increased renal RAS activity in diabetes remains unknown. This increased renal RAS activity is in agreement with the overwhelming clinical evidence that RAS blockers are beneficial in diabetes (Lewis et al., 1993; Haller et al., 2011), most likely in a blood pressure–independent manner (Roksnoer et al., 2016b).
As the majority of patients with CKD are not biopsied, urinary AGT excretion has frequently been used as proxy for intrarenal RAS activity (Table 3). Most (Yamamoto et al., 2007; Nishiyama et al., 2011; Jang et al., 2012; Zhang et al., 2013; Urushihara et al., 2015) but not all (Jang et al., 2014) studies that did compare AGT excretion with biopsy data showed a correlation between kidney Ang II abundance and urinary AGT excretion (often measured in spot urine as urinary AGT to creatinine ratio) (Yamamoto et al., 2007; Nishiyama et al., 2011; Jang et al., 2012; Zhang et al., 2013; Urushihara et al., 2015). In addition, several studies identified a relationship between histologic damage or fibrosis and urinary AGT excretion (Yamamoto et al., 2007; Kim et al., 2011a; Jang et al., 2012; Urushihara et al., 2015). Urinary AGT excretion is the net effect of glomerular filtration, tubular reabsorption, and possibly local release along the nephron (see section II.C). Kidney disease may impact all of these processes. Damage to the glomerular filtration barrier is common in CKD and will increase AGT filtration (Matsusaka et al., 2014; Koizumi et al., 2019). Damage to the proximal tubule or changes in megalin function will alter AGT reabsorption, and impaired AGT reabsorption has been observed in DKD (Tang et al., 2019) and inherited kidney diseases such as Dent’s disease (Roksnoer et al., 2016a) and autosomal dominant polycystic kidney disease (ADPKD) (Salih et al., 2017). Differences in AGT excretion between CKD etiologies can probably be explained by differences in filtration and/or tubular reabsorption (Kobori et al., 2008; Urushihara et al., 2010; Nishiyama et al., 2011; Park et al., 2015b; Salih et al., 2017; Tang et al., 2018; Kim et al., 2019; Ohashi et al., 2020).
TABLE 3
Urinary angiotensinogen in patients with kidney disease
Chronic Kidney Disease |
---|
|
Diabetic Kidney Disease |
|
IgA Nephropathy |
|
Autosomal Dominant Polycystic Kidney Disease |
|
Miscellaneous |
|
ACR, albumin/creatinine ratio; TIN, tubulointerstitial nephritis; UAGT, urinary angiotensinogen.
Urinary AGT is widely used as a marker for intrarenal Ang II generation and therefore intrarenal RAS activity. In almost all patient cohorts with CKD in which urinary AGT excretion was measured, it was closely correlated to urinary albumin excretion (Table 3). Albuminuria is an established predictor for CKD progression and a treatment target, yet whether urinary AGT excretion has added value compared with albuminuria is uncertain. In two studies in patients with DKD (Lee et al., 2017; Ba Aqeel et al., 2019), the predictive effect of urinary AGT excretion on estimated GFR (eGFR) decline was lost after correction for albuminuria. In two cohorts of patients with ADPKD (Kim et al., 2019; Park et al., 2020), AGT excretion was also a predictor of disease progression. However, one study did not include total kidney volume and albuminuria in their model (Kim et al., 2019), and the other study did not correct for known ADPKD risk factors at all (Park et al., 2020). In patients with AKI, AGT and Ang II immunoreactivity in biopsies and urinary AGT excretion were increased and related to AKI severity (Cao et al., 2016). Furthermore, baseline urinary AGT excretion predicted the likelihood of AKI after cardiac surgery (Alge et al., 2013a), in decompensated heart failure (Yang et al., 2015), and in patients admitted to the ICU (Alge et al., 2013b). However, also in these studies, urinary albumin excretion was not included in the models. Given that AGT, like albumin and renin, is filtered by the glomerulus and that all three proteins are subsequently reabsorbed via megalin, with AGT and renin subsequently potentially contributing to renal Ang II generation, it is not surprising that urinary AGT is a proxy for renal RAS activity and future kidney injury. Yet, the same is probably true for urinary albumin. Given that the proximal tubule generates minute amounts of AGT, which are released directly into urine without being converted to Ang II, one possibility is that at a very early stage of CKD/DKD, when filtration/reabsorption are still normal, this AGT already reflects kidney damage prior to any rise in urinary albumin (Saito et al., 2009). Future studies should carefully evaluate this possibility, quantifying urinary and circulating AGT and albumin simultaneously. At later CKD stages, this possibility becomes unlikely since then the contribution of filtered AGT will become overwhelming.
C. Metabolic Disorders
Several studies propose a role for the intrarenal RAS in obesity and insulin resistance. Compared with lean control rats, obese Zucker rats demonstrated decreased renin expression and increased AT2 receptor expression in the kidneys, whereas ACE, AT1 receptor, and Mas receptor expression were unchanged (Ali et al., 2013). Although there were no changes in kidney Ang I and II levels, ACE2 expression and activity was reduced in obese rats. Further, treatment with an AT2 receptor agonist increased renal ACE2 expression and activity, Ang-(1-7) levels, and Mas receptor expression, leading to natriuresis and lower blood pressure (Ali et al., 2013). Conversely, AT1 receptor is increased in obese Zucker rats, whereas treatment with ARBs attenuated the renal expression of inflammatory and fibrosis markers and kidney injury (Xu et al., 2005).
Mice with metabolic syndrome characterized by insulin resistance, visceral obesity, and postprandial hyperglycemia due to a null mutation in carcinoembryonic antigen-related cell adhesion molecule 1 display enhanced expression of RAS components at the level of the renal tubule, resulting in increased renal interstitial fluid Ang II levels (Huang et al., 2013). These mice were also hypertensive and had albuminuria. Notably, all of these abnormalities were exacerbated by high fat intake (Li et al., 2015). Similarly, isolated perfused kidneys from rats treated with a high fructose diet (a model of metabolic syndrome) demonstrate a 2- to 3-fold increase in kidney Ang I and II content without significant differences in Ang-(1-7), ACE, and neprilysin (Yokota et al., 2018). Collectively, these studies suggest that the intrarenal RAS may be activated and could contribute to kidney injury in obesity and insulin resistance.
Consistent with the above notion, multiple clinical studies using ACEIs and ARBs have demonstrated beneficial effects with regard to insulin sensitivity and the development of new-onset diabetes in patients with obesity/metabolic syndrome (Vermes et al., 2003; Tocci et al., 2011). However, it still remains to be determined whether this involves interference with RAS components at the level of the human kidney.
D. In Vitro Models for Human Disease: Kidney Organoids
In vitro models are valuable tools for studying human kidney disease development, progression, and treatment. The increased knowledge on human embryological kidney development and the advance of cell culture technologies have boosted the generation of pluripotent stem cell–derived kidney organoids. These organoids are formed by timely stimulation of Wnt signaling, addition of fibrobast growth factor 9, and 3D-culturing conditions of embryonic stem cells or induced pluripotent stem cells and exhibit remarkable resemblance with first trimester fetal kidney (Morizane et al., 2015; Takasato et al., 2015). Modifications to the extracellular environment can further advance the differentiation status of kidney organoids and drive them into a state that transcriptionally resembles second trimester kidney (Garreta et al., 2019). Morphologically, kidney organoids display glomerular, proximal tubular, and distal tubular structures and contain endothelial cells (Fig. 8).
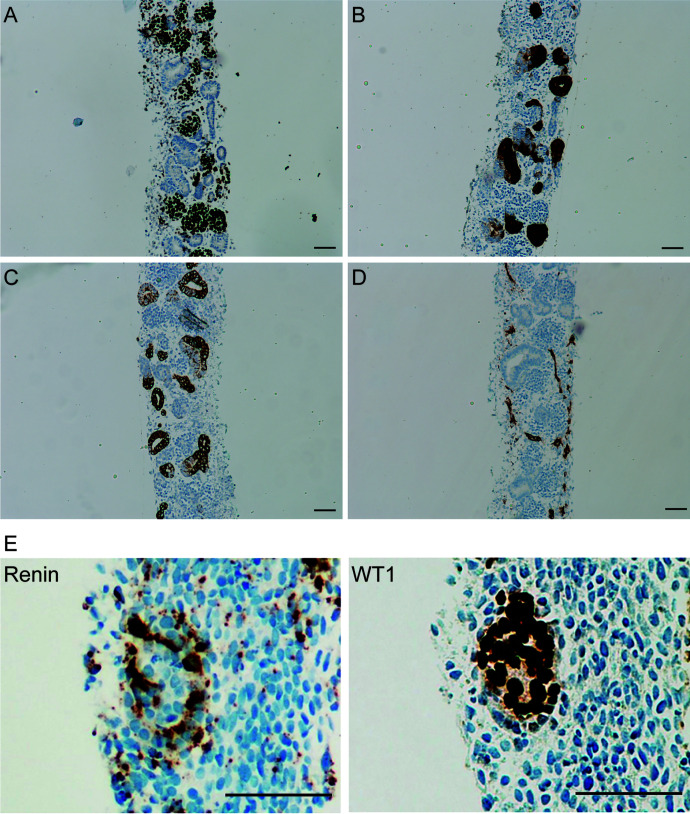
Immunohistochemical characterization of human induced pluripotent stem cell-derived kidney organoids. (A) Wilms’ tumor suppressor gene 1 (WT1) staining of glomerular structures; (B) Villin1 staining of proximal tubular structures; (C) E-cadherin staining of distal tubular structures; (D) CD31 staining of endothelial cells; (E) Renin staining, localized around WT1+ area. Organoids were generated as described by Shankar et al. (2021). Scale bar = 50 μm.
Kidney organoids can be generated from induced pluripotent stem cells derived from kidney disease patients, thereby forming a model to study the impact of genetic disease on kidney development (Forbes et al., 2018). In addition, kidney disease can be modeled in kidney organoids through CRISPR/Cas9 technology. This has been demonstrated by knocking out the polycystic kidney disease genes PKD1 or PKD2, which led to the formation of cysts in organoids (Freedman et al., 2015). The development of automated multiwell kidney organoid culture technology allows for high-throughput toxicity and efficacy screening of novel drugs for treatment of kidney disease (Czerniecki et al., 2018).
Kidney organoids contain a relatively large stromal cell compartment. We recently demonstrated that subsets of stromal cells in kidney organoids have the capacity to produce and secrete enzymatically active renin (Shankar et al., 2021). Renin production could be drastically stimulated by intracellular cAMP elevation and was maintained for at least 2 months after implantation of kidney organoids in a mouse model. Several other components of the RAS are also expressed in kidney organoids, including AGT, AT1 receptor, AT2 receptor, neprilysin, and ACE. Kidney organoids also express ACE2, which sparked research into the role of renal ACE2 in COVID-19. It was demonstrated that SARS-CoV-2 virus can infect kidney organoids and that human recombinant soluble ACE2 can inhibit infection (Monteil et al., 2020; Wysocki et al., 2021). Kidney organoids thus possess properties that allow studies to the RAS in a human in vitro setting, from exploring RAS during kidney development to the impact of disease-specific pluripotent stem cells on RAS and pharmacological intervention.
Although pluripotent stem cells have proven their use for studying in vitro nephrogenesis, adult stem cells derived from human kidney or urine have been demonstrated to be capable of forming kidney tubuloids in a dish. Such tubuloids represent proximal and distal tubular structures and can be propagated in culture for multiple months (Schutgens et al., 2019). As these structures are in a more matured stage of development, they are especially useful for studying kidney repair processes. Tubuloids are likely to express RAS components found in the adult kidney and therefore represent a yet unexplored model for studying pharmacological interventions in the RAS in a human in vitro setting.
V. Renoprotective Drugs Affecting Kidney Angiotensin Levels
A. RAS Inhibitors
Blocking the RAS is currently possible at four levels: renin (renin inhibitors), ACE (ACEIs), AT1 receptors (ARBs), and AGT (siRNA or antisense oligonucleotides), although the latter approach is not yet available in the clinic. The beneficial effects of the ‘classic’ RAS blockers (ACEIs and ARBs) are widely accepted. Figures 9 and and1010 show the changes in the four main angiotensin metabolites, determined by liquid chromatography-tandem mass-spectrometry, after ACEIs, ARBs, AGT siRNA, or their combination in both SHRs and five-sixths nephrectomy rats (Uijl et al., 2019; Bovée et al., 2021). The latter rats display both hypertension and kidney dysfunction. Results in the two models were highly similar.
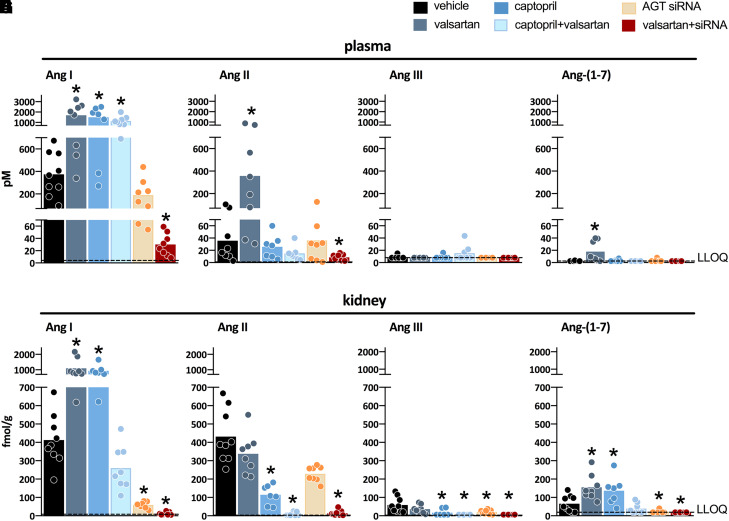
Effect of angiotensin (Ang) II type 1 receptor blockade with valsartan, angiotensin-converting enzyme inhibition with captopril, angiotensinogen (AGT) siRNA, or their combination during 4 weeks on the levels of Ang I, Ang II, Ang III, and Ang-(1-7) in blood and kidney of spontaneously hypertensive rats. Modified from Uijl et al. (2019). *P < 0.05 vs. vehicle. LLOQ, lower limit of quantification.
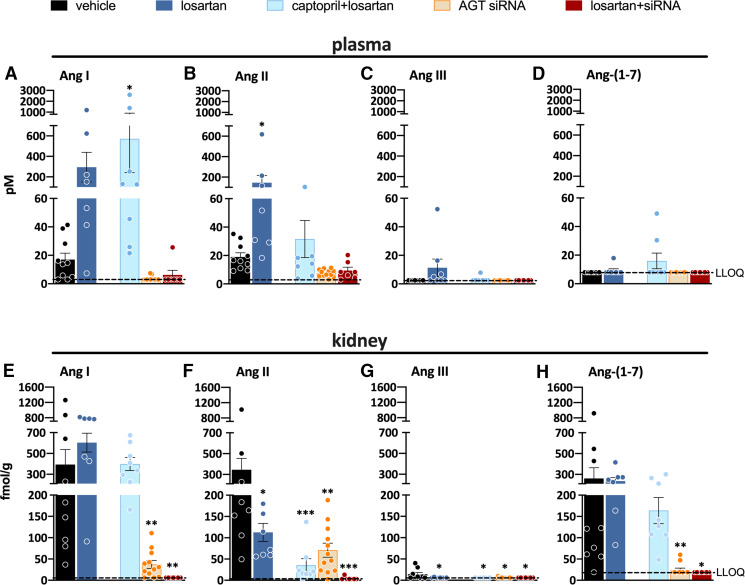
Effect of angiotensin (Ang) II type 1 receptor blockade with losartan, angiotensin-converting enzyme inhibition with captopril, angiotensinogen (AGT) siRNA, or their combination during 4 weeks on the levels of Ang I, Ang II, Ang III, and Ang-(1-7) in blood and kidney of five-sixths nephrectomy rats. Modified from Bovée et al. (2021). *P < 0.05, **P < 0.01, ***P < 0.001 vs. vehicle. LLOQ, lower limit of quantification.
ACEIs lower renal Ang II but not plasma Ang II, both alone and in combination with an ARB. ACEIs increase Ang I in both kidney and blood, and apparently this is sufficient to restore circulating Ang II but not renal Ang II. This indicates that the kidney is very sensitive to ACE inhibition, as has been suggested before, also in comparison with other organs (Campbell et al., 1995b; Campbell, 1996). The observation that the Ang II/I ratio (the best in vivo indicator of ACE activity) dropped by >90% after ACEIs argues against a significant contribution of other converting enzymes in the kidney. Here we need to consider that ACE inhibition in vivo is unlikely to reach 100%, and thus the remaining <10% renal Ang I-II converting activity likely reflects the small fraction of ACE that is not inhibited (i.e., it does not by definition support a role for other converting enzymes like chymase). These data also indicate that modest ACE upregulation, even if occurring, would have marginal consequences. This is not surprising since the blockade of >90% will also apply to any de novo synthesized ACE. To truly overcome such blockade, 10- to 20-fold rises in ACE rises are required. In reality, ACE upregulation is usually in the order of 2-fold or less (Gonzalez-Villalobos et al., 2013). The only RAS component capable of matching a >90% blockade is renin, allowing immediate and substantial upregulation of Ang I and therefore ongoing Ang II generation even when only <10% of ACE activity is left. A limit in its capacity to rise has not yet been described, and rises of several 100-fold are easily feasible (Balcarek et al., 2014). This is related to the fact that VSMCs along the renal arterioles can transform into renin cells (see section II.A). Hence, depending on the ACEI dose and the degree of renin upregulation, which may differ per condition (e.g., renin rises are usually more substantial during a low-salt diet), it is possible that occasionally renal Ang II levels do return to normal after long-term ACEI treatment. If so, the question arises as to why ACE inhibition would be effective at all. Here one might argue that Ang II generation normally occurs in a highly localized manner and is followed by its rapid binding to surrounding AT receptors to exert effects. After ACEI treatment, rapidly reaching high local Ang II levels is no longer possible. Higher renin levels, combined with modest ACE upregulation at additional sites, may help to restore the total amount of Ang II that is generated. However, the generation now occurs more evenly spread and no longer regionally, and thus AT1 receptor stimulation occurs less efficiently (Fig. 11).
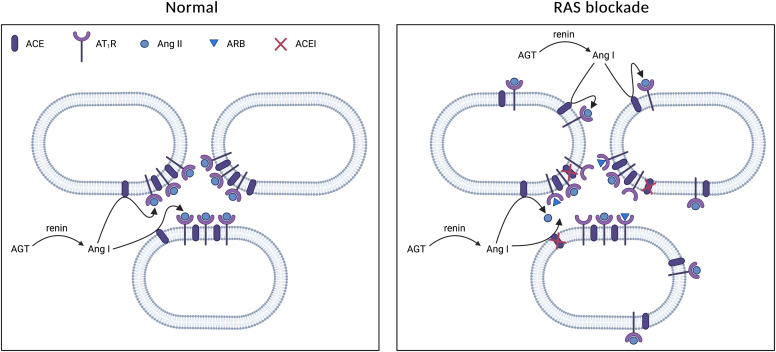
Proposed mechanism of how renin-angiotensin system (RAS) blockers work, even when not lowering the tissue levels of angiotensin (Ang) II. Normally, Ang II generation occurs in a highly localized manner by angiotensin-converting enzyme (ACE), allowing local Ang II type 1 receptor (AT1R) stimulation. After treatment with an AT1R (ARB) or ACE inhibitor (ACEI), this is no longer possible, although upregulation of ACE and AT1R expression at alternative sites may occur, thus still allowing Ang II formation and AT1R stimulation but in a less efficient (‘diluted’) manner.
Data on the effect of renin inhibition on renal tissue Ang I and II levels are scarce. This is related to the fact that the only renin inhibitor that is currently clinically available, aliskiren, is a human renin inhibitor with limited efficacy for rodent renin. Moreover, its oral bioavailability is very low (Danser, 2007). Unfortunately, renin, unlike ACE and the AT1 receptor, is highly species specific, and thus ACEIs and ARBs can be easily tested in rodents whereas renin inhibitors cannot, except of course in rodents that are transgenic for human renin and AGT (Pilz et al., 2005). Theoretically, one would expect a renin inhibitor to lower Ang II (and Ang I) even better since such an inhibitor would also prevent the consequences of the rises in renin that will occur during RAS blockade. Although aliskiren did indeed lower Ang I and II acutely in the SHR and Sprague Dawley kidney (van Esch et al., 2010a; Campbell et al., 2011), the fact that only very high doses were tested, whereas no information was obtained on the changes in the other angiotensin metabolites, precludes clear conclusions on its efficacy with regard to renal angiotensin levels. The limited evidence that is available supports that aliskiren is at least as renoprotective as other RAS blockers, both in animals and in humans (Pilz et al., 2005; Fisher et al., 2008; van Esch et al., 2010a; Campbell et al., 2011).
Figures 9 and and1010 show that ARBs increase circulating Ang I and II to the same degree, while not affecting renal Ang II, and at most increase renal Ang I. Hence, the renal Ang II/I ratio usually decreases substantially after ARB treatment. These data reflect the well known rise in RAS activity that will occur after ARB treatment. Yet, although there is much more Ang II, it cannot bind to its receptors at renal tissue sites due to the fact that the receptors are now largely occupied by the ARB. Thus, a high degree of local AT1 receptor stimulation is no longer achievable, and at most the total tissue Ang II levels remain the same, but this Ang II is now more evenly spread (Fig. 11). Ang II may also bind to its AT2 receptor and exert anti-AT1 receptor effects (see section III.B).
In blood, both Ang-(1-7) and Ang III remained low or undetectable during ACEI and ARB treatment (Figs. 9 and 10). The same is true for Ang III in the kidney, the level of which, if anything, decreased even further during treatment. This raises doubt about the concept that Ang III is the endogenous agonist of the AT2 receptor (Padia et al., 2008; van Esch et al., 2008). In SHRs, renal Ang-(1-7) levels increase during ACEI and ARB treatment, in parallel with Ang I, but this was not seen in the five-sixths nephrectomy rat, where Ang-(1-7) levels remained unchanged. Nevertheless, this implies that during both types of treatment the Ang-(1-7)/Ang II ratio generally increased, favoring effects of the former (see section III.C).
As discussed in section II.C, the use of AGT siRNA has demonstrated that renal angiotensin generation depends on hepatic AGT. Remarkably, despite lowering plasma AGT by >95%, siRNA did not alter blood Ang I or II in SHRs, whereas after siRNA only a modest drop in circulating Ang II was seen in the five-sixths nephrectomy model. Yet, at the level of the kidney, all angiotensin metabolites went down after siRNA in both models, and in combination with an ARB they quite often almost entirely disappeared (Figs. 9 and 10). The drop in renal tissue Ang II directly translated into a reduction in proteinuria in the five-sixths nephrectomy rat (Bovée et al., 2021). In blood, dual RAS blockade also lowered Ang II but not as completely as in the kidney. These data therefore illustrate the great sensitivity of the kidney to AGT suppression. This is probably related to the fact that the renal AGT supply relies entirely on a complicated AGT uptake process, depending on (normally) very high circulating AGT levels in the Michael-Menten constant (Km) range. Dropping these by >95% hampers sufficient uptake and will affect the possibility of generating sufficient angiotensins at tissue sites. A similar situation has been observed in subjects with end-stage heart failure receiving left ventricular assist device support (Klotz et al., 2009). Their renin levels were so high (immediately cleaving the small quantities of AGT that were still left) that cardiac AGT became depleted, thus no longer allowing Ang II generation at cardiac tissue sites. The fact that a >95% drop in AGT still allowed circulating Ang II levels to be in the normal range is yet another example of the versatility of the RAS to restore its activity, at least in blood. This relied on a very substantial rise in renin and thus made the rat circulating RAS look temporarily like the mouse circulating RAS (which is characterized by AGT levels in the order of a few percent of those in humans and rats).
Finally, although it was initially believed that the more RAS blockade the better in patients who require RAS blocker treatment, this turned out not to be true (Parving et al., 2012; Fried et al., 2013). Too much RAS blockade resulted in hyperkalemia, hypotension, and renal failure (i.e., the predictable consequences of RAS annihilation), especially when renal function was already compromised when the additional blocker was added. The beneficial effects did not increase concomitantly. This outcome is due to the fact that kidney function (particularly glomerular filtration) depends on the RAS (Balcarek et al., 2014). Hence, a patient requires optimal rather than maximal RAS blockade (Danser and van den Meiracker, 2015). This does not imply that the use of more than one RAS blocker should now always be avoided: it may still be needed to obtain sufficient RAS suppression in an individual patient.
A condition in which RAS blockers should not be used is pregnancy. This is because the RAS is needed for the development of the fetal kidney since Ang II acts as an important growth factor (e.g., for proximal tubular cells) (Tufro-McReddie et al., 1994). Indeed, AGT KO mice display renal morphologic abnormalities such as tubulointerstitial lesions and papillary atrophy, resulting in an impaired urine-concentrating ability and low blood pressure (Niimura et al., 1995; Kihara et al., 1998). Similarly, mutations in RAS genes (renin, AGT, ACE, and AT1 receptor) resulting in the absence or inefficacy of Ang II associated with autosomal recessive renal tubular dysgenesis, hypotension, and anuria (Gribouval et al., 2005; Richer et al., 2015).
B. Soluble ACE2
An alternative approach to deal with excessive renal Ang II levels is to increase its degradation by amplifying ACE2 activity (Wysocki et al., 2019; Marquez et al., 2021). This is particularly relevant given the decreased ACE2 activity in several kidney pathologies, as discussed in section II.E. Different approaches have been used to amplify ACE2 activity to achieve a therapeutic benefit or as proof-of-concept studies. This includes viral delivery systems (lenti-, adeno- and adeno-associated virus), minicircle DNA delivery, and the administration of recombinant proteins (Marquez et al., 2021). Here, we briefly mention the therapeutic potential of recombinant ACE2 proteins, focusing on kidney disease and COVID-19.
The therapeutic benefit of ACE2 amplification presumably depends on both the dissipation of excess Ang II and the formation of Ang-(1-7) (Fig. 12). Wysocki et al. (2010) demonstrated the effectiveness of both human and mouse recombinant soluble ACE2 in Ang II–dependent hypertension (Ye et al., 2012). To accomplish this potential therapeutic action, both the native recombinant soluble ACE2 protein (740 amino acids) and the full-length ACE2 gene have been used. In STZ-induced diabetes, overexpression of human ACE2 in podocytes increased podocyte numbers and delayed the onset of albuminuria (Nadarajah et al., 2012). In a rat model of STZ-induced diabetes, ACE2 expression by adenovirus reduced systolic blood pressure, urinary albumin excretion, and creatinine clearance and improved the glomerulosclerosis index (Liu et al., 2011a). In STZ-treated diabetic mice, both minicircle DNA gene delivery of soluble ACE2 and intraperitoneal injection of recombinant mouse soluble ACE2 protein increased plasma ACE2 levels; however, there was no effect on the hyperfiltration typical of early diabetes or glomerular lesions (Wysocki et al., 2017b). The large molecular size of the native soluble protein was considered the limiting factor when targeting the kidney directly since kidney and urinary ACE2 levels were not increased after injection of recombinant ACE2/minicircle DNA delivery of soluble ACE2 in these mice (Wysocki et al., 2013, 2017b). Conversely, in Col4a3 KO mice, a model of experimentally induced Alport syndrome, urinary ACE2 activity increased markedly after injection of recombinant mouse ACE2 (Wysocki et al., 2017b). Moreover, this injection improved kidney fibrosis (Bae et al., 2017). Systemically administered native recombinant ACE2 normally cannot cross the glomerular filtration barrier due to its large molecular size (120–130 kDa). Yet, in this model of Alport syndrome it does pass through, owing to the markedly disrupted glomerular filtration barrier (Wysocki et al., 2017b). To overcome this limitation for treatment of early kidney disease, novel recombinant soluble mouse ACE2 proteins of smaller molecular size (605 to 619 amino acids) were developed (Wysocki et al., 2019). These shorter truncates are systemically active and amenable to glomerular filtration as shown by increased kidney ACE2 activity in ACE2 KO mice after ACE2 1-619 infusion, whereas there was no increase in animals infused with native ACE2 1-740 (Wysocki et al., 2019). To increase the half-life of the shorter ACE2 truncates, Wysocki et al. (2021) fused a human counterpart of ACE2 with an albumin binding domain. The resulting fusion protein has a markedly extended half-life and is now being tested in preclinical models of kidney disease and COVID-19.
Given that ACE2 is the main cell entry receptor for SARS-CoV-2, there may be a role for soluble ACE2 proteins to act as a decoy in COVID-19 (Batlle et al., 2020b; Wysocki et al., 2021; Hassler et al., 2022), preventing viral internalization. A consequence of SARS-CoV-2 infection is internalization of ACE2, which causes a decrease in membrane-bound ACE2 (Kuba et al., 2005). This should result in loss of enzymatic ACE2 activity, which would favor the accumulation of proinflammatory peptides like Ang II, thereby increasing the risk of local injury. Indeed, in ACE2 KO mice, acid aspiration–induced lung injury was more severe than in wild-type mice (Imai et al., 2005). The enzymatic action of administered soluble ACE2 proteins, in addition to the decoy effect, might help to prevent accumulation of these harmful peptides (Monteil et al., 2020; Wysocki et al., 2021; Hassler et al., 2022).
The decoy effect of several soluble ACE2 proteins has now been tested in kidney organoids and animal models of SARS-CoV-2 infection (Monteil et al., 2020; Wysocki et al., 2021; Hassler et al., 2022). A soluble ACE2 variant with increased binding affinity for SARS-CoV-2 infection prevented severe disease, lowered viral lung and brain titers and markedly improved lung histology in k18hACE2 mice, a model of otherwise lethal SARS-CoV-2 infection (Hassler et al., 2022). The kidneys in this model of SARS-CoV-2 infection, however, are not well studied, but AKI is a complication frequently seen in patients with severe COVID-19 (Batlle et al., 2020a). In the aforementioned study, some k18hACE2 mice 6 days post–SARS-CoV-2 infection did develop severe proximal tubular injury, based on histologic findings, whereas soluble ACE2 markedly improved kidney histology (Hassler et al., 2022). The only clinical trial based on the systemic administration of a native soluble ACE2 protein approved for clinical use in COVID-19 patients did not meet the primary endpoints, a composite of all-cause death or invasive mechanical ventilation (NCT04335136). One possibility for these results is that the systemic route is likely not as effective as the intranasal route to neutralize SARS-CoV-2, and in addition the short duration of action of this protein may not afford 24-hour protection to intercept SARS-CoV-2 from getting into cells.
C. Neprilysin Inhibitors: ARNI
Dual ARNI is currently registered for the treatment of patients with heart failure (Ponikowski et al., 2016), in whom studies showed a substantial reduction in mortality and hospitalization (McMurray et al., 2014). Sacubitril/valsartan (LCZ696) is the first-in-class ARNI. In vivo, sacubitril is rapidly metabolized into the active neutral endopeptidase/neprilysin (NEP) inhibitor sacubitrilat. NEP degrades both vasodilators (e.g., natriuretic peptides, bradykinin, adrenomedullin) and vasoconstrictors (e.g., Ang II, endothelin-1). Therefore, the effect of single NEP inhibition is unpredictable, as it depends on the dominance of either vasodilators or vasoconstrictors (Ando et al., 1995). Dual therapy, however, guarantees the beneficial effects of increasing natriuretic peptides (e.g., increased diuresis, natriuresis, and vasodilation) because simultaneous blockade of the AT1 receptor with valsartan counteracts the NEP inhibitor–induced rise in Ang II.
Recent studies have investigated whether sacubitril/valsartan might also be renoprotective. When compared with conventional RAS inhibitors, sacubitril/valsartan slowed the rate at which kidney function declined in patients with heart failure, both in those with and without concurrent CKD (Voors et al., 2015; Damman et al., 2018; Mc Causland et al., 2020). Unexpectedly, sacubitril/valsartan-treated patients displayed a slight increase in urinary albumin-creatinine ratio (Voors et al., 2015; Damman et al., 2018). However, baseline albuminuria was very low in these studies, and sacubitril/valsartan may reduce proteinuria particularly in patients with macroalbuminuria (Ito et al., 2015). In contrast, in the United Kingdom Heart and Renal Protection-III trial, sacubitril/valsartan had similar effects to irbesartan on both measured GFR and albuminuria in patients with moderate-to-severe CKD, even though blood pressure control and neurohumoral markers of cardiac dysfunction were improved superiorly (Haynes et al., 2018). CKD was caused by diabetes in only a subset of the participants, whereas sacubitril/valsartan appears to be most effective at protecting kidney function in this population (Packer et al., 2018). Indeed, in rats with severe diabetic, hypertensive kidney damage, ARNI induced a greater reduction in proteinuria and glomerulosclerosis than ARB (Roksnoer et al., 2016c). This appeared to be due to preserved podocyte integrity and was independent of blood pressure changes (Uijl et al., 2020). It involved the upregulation of atrial natriuretic peptide, which increased nephrin expression and suppressed both transient receptor potential canonical 6 and regulator of calcineurin 1. Superior renoprotective effects of sacubitril/valsartan versus valsartan were also seen in obese Zucker rats and the five-sixths nephrectomy model (Jing et al., 2017; Habibi et al., 2019).
A critical question remains as to whether the ARNI effects are simply the added consequence of blocking AT1 receptors and increasing natriuretic peptide levels or if they involve an additional (synergistic?) interaction between the two systems (e.g., in the kidney). Natriuretic peptides directly suppress renin release from JG cells (Henrich et al., 1988). Furthermore, if NEP inhibition truly increases Ang II levels, this should also suppress renin. Currently, knowledge on this topic after ARNI treatment is entirely lacking. In a study in transgenic (mRen2)27 rats (i.e., hypertensive rats that overexpress the mouse Ren2 gene), NEP inhibition did not affect renin (Roksnoer et al., 2015). This could be due to the fact that in these rats, renin not only originates in the kidney. In human kidney homogenates, NEP has been suggested to generate Ang-(1-7) from Ang I (Kaltenecker et al., 2020). Remarkably, NEP levels were diminished in CKD kidneys compared with healthy kidney donors, implying that Ang-(1-7) would be low in CKD. Assuming that Ang-(1-7) exerts a beneficial effect, this finding would argue against the use of NEP inhibition in such patients, in full contrast with the abovementioned clinical trials. Here it is important to stress that data obtained in homogenates are unlikely to represent in vivo physiology. Indeed, treatment of rats with a NEP inhibitor did not alter renal Ang-(1-7) levels, neither at baseline nor on top of ARB treatment (Roksnoer et al., 2015). Thus, in reality, the in vivo contribution of NEP to Ang I-Ang-(1-7) conversion may be negligible. Taken together, the evidence obtained until now does not favor a synergistic interaction between AT1 receptor antagonism and NEP inhibition at the level of the kidney.
D. Cyclooxygenase Inhibitors
Prostaglandins are produced from arachidonic acid by COX-1 and -2 in combination with terminal prostaglandin synthases. In the kidney and its vasculature, both COX-1 and COX-2 are present. COX-2 activity is further inducible by changes in salt intake and RAS activity with a differential regulation between cortex and medulla (Fig. 13) (Yang et al., 1998). PGE2 is the most abundantly produced prostaglandin in the kidney. PGE2 plays an important role in the regulation of renin release, kidney blood flow, tubular water and salt handling, kidney development, and the proliferative response to injury. COX-1 and COX-2 are inhibited by nonsteroidal anti-inflammatory drugs (NSAIDs). Selective inhibitors of COX-2 were developed to avoid the gastrointestinal side effects of NSAIDs. Both NSAIDs and COX-2 inhibitors are associated with increased cardiovascular mortality, hypertension, and nephrotoxicity, especially in hypovolemic subjects (Bhala et al., 2013). Interestingly, COX inhibition can also be renoprotective in hyperfiltrating and albuminuric CKD patients by reducing glomerular perfusion pressure, with similar short-term benefits as RAS inhibition (Vogt et al., 2009). To further mitigate the systemic side effects of COX inhibition, specific PGE2 (EP) receptor agonists and antagonists are currently being developed for different indications and used in animal models of kidney disease and hypertension (Markovič et al., 2017). In the kidney, the effects of the COX-PGE2 axis and the RAS are closely linked in regulating renovascular resistance, renin secretion, and sodium excretion (Nasrallah et al., 2014).
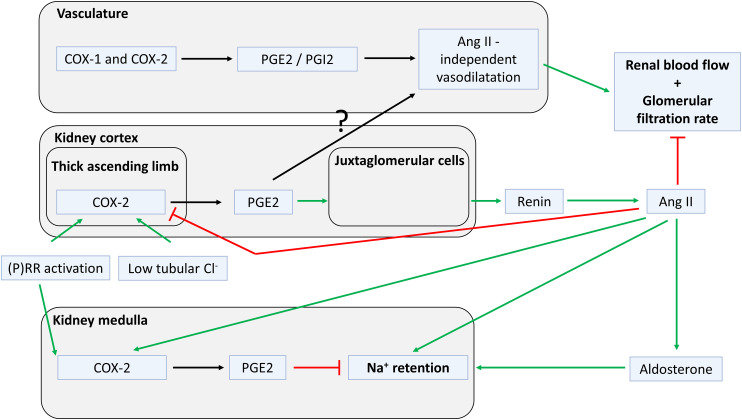
Interactions between prostaglandins and the renin-angiotensin system (RAS) in the kidney. Firstly, in the vasculature, prostaglandin E2 (PGE2) and I2 (PGI2) cause vasodilatation, counteracting the vasoconstriction of angiotensin (Ang) II. Secondly, in the kidney cortex, PGE2 is produced when hypovolemia occurs and subsequently induces renin production by juxtaglomerular cells. Finally, in the kidney medulla, PGE2 counteracts the effects of Ang II on sodium retention.
In the glomerulus, COX-1–derived PGI2 and PGE2 cause vasodilation of the afferent arteriole and maintain kidney blood flow and GFR in situations with active vasoconstrictors through activation of the EP4 receptor (Tang et al., 2000). Even though the vasodilatory effect of PGE2 depends on concurrent vasoconstriction by other mediators, the effect of COX inhibition on renovascular flow is independent of intrarenal Ang II. This was shown in a study that compared two groups of rats with similar cortical COX activity but different Ang II levels (Green et al., 2010). Rats on a low-sodium diet had increased cortical COX and kidney Ang II levels, whereas rats treated with ACEIs had increased cortical COX activity but low kidney Ang II levels. After COX inhibition, eGFR and kidney blood flow were reduced similarly in both groups, providing evidence for the independence of PGI2 and PGE2 effects on vascular tone from prevailing RAS activity (Green et al., 2010).
The COX-PGE2 axis and its interaction with the RAS are also essential for normal kidney development. COX-2 KO mice and neonatal mice treated with COX-2 inhibitors develop kidney failure characterized by cortical damage, with immature glomeruli and tubules (Dinchuk et al., 1995; Morham et al., 1995). Kidneys from human fetuses exposed to NSAIDs show similar histologic defects (van der Heijden et al., 1994; Morham et al., 1995; Antonucci et al., 2012). These developmental defects may partly be mediated by a reduction in RAS activity. During normal kidney development, changes in COX-2 and renin mRNA levels occur concurrently, related to the fact that COX-2–derived PGE2 stimulates renin release, whereas AT1 receptor stimulation subsequently suppresses COX-2. Indeed, COX-2 KO mice do not show the postnatal rise in renin that is essential for normal kidney development (Kaplan et al., 1994). Furthermore, part of the histologic damage in COX-2 KO mice was ameliorated by treatment with an ARB (Kaplan et al., 1994), again illustrating the close COX-PGE2-RAS interaction during kidney development.
The interaction between COX and PGE2 in the kidney medulla is different from its regulation in the kidney cortex and during kidney development. Medullary COX-2 is important for renin release by JG cells. After binding its EP2 or EP4 receptor, PGE2 produced by COX-2 in the thick ascending limb stimulates renin exocytosis from JG cells (Fig. 13) (Schweda et al., 2004). COX-2 expression in the thick ascending limb is regulated by the intratubular chloride concentration, which is sensed by the sodium-potassium-chloride cotransporter 2 (Harris et al., 2004). Dietary salt restriction, diuretics, and ACEIs all increase cortical COX-2 expression and PGE2 production (Harris et al., 1994; Cheng et al., 2002). In isolated perfused tubules, COX-2 inhibition prevented the increase in renin release by low chloride concentrations (Traynor et al., 1999). The increase in renin by dietary salt restriction or ACEIs was also reduced in vivo by COX-2 inhibition and in COX-2 KO mice (Kim et al., 2007). In patients with salt-losing tubulopathies who have high circulating renin levels, cortical COX-2 expression is increased (Kömhoff et al., 2000) and inhibition of COX-2 reduces circulating renin (Blanchard et al., 2015). Similar increases in COX-2 expression have been observed in patients with diabetes and in patients with heart failure (Khan et al., 2001).
Ang II suppresses cortical COX-2 via its type 1 receptor, even at nonpressor doses, and AT1 receptor–deficient mice have increased COX-2 expression (Cheng et al., 1999; Zhang et al., 2006). Conversely, activation of the AT2 receptor increases cortical COX-2 expression; the net effect of Ang II, however, is a reduction of cortical COX-2 activity (Zhang et al., 2006). As discussed earlier, a link between the (P)RR and COX-2 has also been reported (Kaneshiro et al., 2006; Riquier-Brison et al., 2018). Human (P)RR overexpression increases cortical COX-2 expression in mice, and COX-2 inhibition diminishes kidney blood flow in these animals without a difference in kidney Ang II levels (Kaneshiro et al., 2006). Another study confirmed that (P)RR siRNA and macula densa–specific (P)RR KO reduce COX-2 expression, PGE2 generation, plasma renin, and blood pressure (Riquier-Brison et al., 2018).
In the kidney, medullary COX-2 expression is upregulated by a high-sodium diet (Yang et al., 1998), and PGE2 produced by COX-2 protects against salt-sensitive hypertension by increasing sodium excretion (Zhang et al., 2018) (Fig. 13). This natriuretic effect of COX-2 is stronger in rats with increased RAS activity (Green et al., 2010). In human subjects treated with NSAIDs or COX-2 inhibitors, a transient decrease in sodium excretion with an associated increase in blood pressure or edema is frequently observed (Catella-Lawson et al., 1999). PGE2 production in the kidney medulla is not only increased by high dietary sodium intake but also directly by Ang II. Mice increase medullary COX-2 expression after Ang II infusion (Gonzalez et al., 2014a), and this effect is diminished in mice with CD-specific AT1 receptor deletion (Stegbauer et al., 2017). Such KO mice display a stronger increase in blood pressure after Ang II infusion (Stegbauer et al., 2017). Inhibiting either COX-2 or the EP4 receptor also enhances the effect of Ang II infusion on blood pressure (Qi et al., 2002), illustrating the important role of COX-2 and PGE2 in limiting the Ang II–induced sodium retention in the CD. (P)RR activation by prorenin also stimulated COX-2 activity in cortical and in medullary CD cell lines (Gonzalez et al., 2013; Salinas-Parra et al., 2017). ERK1/2 inhibition and short hairpin RNA interfering with (P)RR both prevented this effect, and it did not involve Ang II formation and AT1 receptor activation. Moreover, PGE2 increased (pro)renin synthesis in CD cells. The hypothesis is that the upregulation of COX-2 and increased PGE2 production by CD prorenin could counteract some of the effects of Ang II on sodium retention by increasing medullary blood flow and sodium excretion. However, in vivo evidence for this concept is lacking.
E. Sodium-Glucose Cotransporter-2 Inhibitors
Hyperglycemia induces oxidative stress and inflammation, thus activating the intrarenal RAS (Nishiyama and Kobori, 2018). Subsequently, this renal RAS activation induces hypertension, renal hemodynamic changes, and renal injury, making RAS inhibitors a logical choice to lower blood pressure and slow the progression of kidney injury in patients with DKD. SGLT2 inhibitors were developed as hypoglycemic agents that increase glycosuria by inhibiting reabsorption of filtered glucose within proximal tubules. Yet, recent clinical trials have shown that SGLT2 inhibitors significantly decrease the risk of kidney injury in both diabetic and nondiabetic patients with CKD on top of RAS inhibitors (Zelniker et al., 2019; Cherney et al., 2020). The mechanisms underlying the beneficial effects of SGLT2 inhibitors on kidney outcomes remain unclear. Miyata et al. (2021) recently showed that administration of a SGLT2 inhibitor attenuated glomerular sclerosis and tubulointerstitial fibrosis in Ang II–treated mice independently of changes in blood pressure and GFR. These data suggest a potential role of the intrarenal RAS in the renoprotective effects of SGLT2 inhibitors (Satou et al., 2020).
SGLT2 inhibitors cause reductions in body fluid and blood pressure (Rahman et al., 2017). Thus, it has been proposed that renin release may be activated after administration of SGLT2 inhibitors (Nishiyama and Kobori, 2018). Indeed, several animal studies showed that treatment with SGLT2 inhibitors led to increased plasma renin activity in both diabetic mice (Woods et al., 2019) and nondiabetic CKD rats (Li et al., 2018a), whereas other studies observed no such change (Shin et al., 2016). In patients with type 2 diabetes, SGLT2 inhibitors increased plasma renin, plasma aldosterone, and urinary aldosterone (Eickhoff et al., 2019). Plasma renin also increased in patients with acute heart failure treated with SGLT2 inhibitors (Boorsma et al., 2021). However, plasma aldosterone levels were not affected by SGLT2 inhibitors in nondiabetic healthy volunteers and in patients with acute heart failure (Amin et al., 2015; Boorsma et al., 2021). These contradictory results may be explained by the diuretic properties of SGLT2 inhibitors. Although administration of SGLT2 inhibitors transiently induces diuresis and negatively impacts water balance in the body (Ansary et al., 2017), continuous administration of SGLT2 inhibitors minimizes daily changes in fluid balance (Yasui et al., 2018). Possible effects of SGLT2 inhibitors on sympathetic nerve activity (Wan et al., 2018) may also influence renin release from JG cells.
Treatment with SGLT2 inhibitors significantly lowered urinary AGT levels and renal AGT expression in animal models of type 2 diabetes (Woods et al., 2019), and a similar tendency was observed in patients with type 2 diabetes (Yoshimoto et al., 2017). In contrast, in patients with type 1 diabetes (Cherney et al., 2014) and obese rats fed a high-salt diet (Takeshige et al., 2016), SGLT2 inhibition increased urinary AGT levels. On the other hand, treatment with SGLT2 inhibitors did not alter renal AGT expression in a mouse model of type 1 diabetes (Li et al., 2018a). Thus, the effects of SGLT2 inhibitors on intrarenal AGT remain controversial. However, it is important to note that the intrarenal RAS is likely activated in subjects with diabetes, and one possibility is that the effect of SGLT2 inhibitors relies on the basal activity of the system. Moreover, diabetic and nondiabetic patients with CKD are usually already treated with RAS inhibitors (Heerspink et al., 2020), which are likely to coinfluence the intrarenal RAS.
As discussed in section II.C, the liver is the main source of intrarenal AGT, especially in the setting of proteinuria (Matsusaka et al., 2014). However, AGT is also expressed within proximal tubules and released into urine (Nishiyama and Kobori, 2018). Thus, it is important to unravel the sources of AGT in urine during treatment with SGLT2 inhibitors. Clinical studies have shown that treatment with SGLT2 inhibitors significantly decreases albuminuria in patients with CKD (Heerspink et al., 2020). Consequently, glomerular filtration of circulating AGT may also be reduced by SGLT2 inhibitors, although currently no data support this.
Exposure to high glucose significantly increased AGT mRNA expression in cultured human proximal tubular cells (Wang et al., 2017a). Therefore, a reduction in intracellular glucose levels after treatment with SGLT2 inhibitors may decrease AGT generation in proximal tubular cells. Indeed, treatment with SGLT2 inhibitors or an SGLT2 short hairpin RNA attenuated upregulation of AGT expression after exposure of cultured mouse proximal tubular cells to high glucose (Satou et al., 2020). In diabetic New Zealand obese mice fed a high-fat diet, high levels of AGT expression in renal tissue were decreased after treatment with an SGLT2 inhibitor (Woods et al., 2019). It is important to emphasize, however, that SGLT2 inhibition can increase glucose delivery to the distal proximal tubule (S3 segment), and therefore AGT production at this site may be stimulated. Thus, different effects of SGLT2 inhibitors on AGT production at early and late proximal tubules may lead to inconsistent conclusions regarding responses of intrarenal RAS to SGLT2 inhibition. Indeed, some studies have found that SGLT2 inhibitors lower renal tissue AGT expression (Han et al., 2018), whereas others have shown the opposite (Wang et al., 2017b) using the same diabetes mouse model. The potential mechanisms through which SGLT2 inhibitors may influence the intrarenal RAS are illustrated in Fig. 14.
VI. Conclusions
Local angiotensin formation in the kidney is a rapidly evolving field, which started many years ago as an ‘independent system’ relying on AGT synthesis in the proximal straight tubule and prorenin synthesis in the CD. The latter would act on/via the (P)RR, and urinary AGT and prorenin would then reflect renal RAS activity. In reality, we now know that angiotensin generation at renal tissue sites fully depends on AGT of hepatic origin, whereas CD (pro)renin rather reflects uptake of filtered urinary (pro)renin that has not yet been reabsorbed. Megalin turns out to be a novel player, reabsorbing both AGT and (pro)renin, and consequently, the urinary levels of these RAS components largely reflect the net effect of glomerular filtration and megalin-mediated tubular reabsorption. Since both filtration and megalin expression are altered in disease conditions (like DKD), this will directly affect the urinary levels of AGT, renin, and prorenin, thus explaining why their levels correlate with disease severity. In fact, megalin-dependent AGT reabsorption may underlie renal angiotensin generation, particularly after podocyte injury, which will greatly increase glomerular leakage of plasma AGT. Under healthy conditions, renal Ang II is probably generated from liver-derived AGT in the capillary lumen or interstitium since filtration/reabsorption then does not yield sufficient AGT. Given that renal angiotensin generation depends on hepatic AGT, it is not surprising that liver-targeted AGT siRNA and antisense oligonucleotides effectively suppress the renal RAS and may thus exert renoprotection in a blood pressure–independent manner.
Megalin-mediated reabsorption depends on the (P)RR, but this receptor as well as its soluble variant s(P)RR now turn out to have a wide variety of effects on kidney function (water transport, sodium reabsorption) and fibrosis that are unrelated to the RAS. Hence, (P)RR-(pro)renin interaction underlying multiple renal effects currently is a less likely scenario. Nevertheless, this implies that interfering with the (P)RR might offer beneficial effects on top of RAS blockade. The major effector of the RAS remains the AT1 receptor, with possible modulatory (counteracting) roles for both the AT2 receptor and Mas receptor. Here an urgent question is what agonist activates these latter two receptors since generally the preferred agonists of the AT2 receptor (Ang III) and the Mas receptor [Ang-(1-7)] occur at much lower levels than those of Ang II, if detectable at all. This may differ under conditions where RAS blockers are introduced or after the application of soluble ACE2, which converts Ang II to Ang-(1-7). Since membrane-bound ACE2 is simultaneously the main cell entry receptor for SARS-CoV-2, its application as a soluble variant may function as a decoy, preventing cellular accumulation of the virus. Kidney organoids would allow us not only to study this concept in a human in vitro setting but also to explore novel and existing drugs. ARNI and SGLT2 inhibitors have now entered the clinic and are currently being studied closely because of their renoprotective effects. This may involve interference with the renal RAS, although neprilysin inhibition alone does not appear to alter renal angiotensin generation. Finally, COX inhibition may reemerge as a renoprotective approach in hyperfiltrating and albuminuric CKD patients by reducing glomerular perfusion pressure, with similar short-term benefits as RAS inhibition. However, given the systemic side effects of COX inhibition, a more likely scenario is interfering at the level of one or more of the prostaglandin receptors, in particular those of PGE2, which are linked to both renin synthesis and the (P)RR. Taken together, local synthesis of renin and prorenin at the site of the JG apparatus, with the capacity of an upregulation of several 100-fold by transforming VSMCs along the renal arterioles into renin cells, combined with megalin-mediated uptake of hepatic AGT and the occurrence of a wide variety of angiotensinases and AT receptors, allow the kidney to possess a local RAS that acts independently of the circulating RAS. A wide variety of existing and novel drugs exert beneficial renal effects by interfering with this intrarenal RAS.
Abbreviations
ACE | angiotensin-converting enzyme |
ACEI | ACE inhibitor |
ADAM | a disintegrin and metalloprotease |
ADPKD | autosomal dominant polycystic kidney disease |
AGT | angiotensinogen |
Ang | angiotensin |
APA | aminopeptidase A |
ARB | AT1 receptor blocker |
ARNI | angiotensin receptor/neprilysin inhibition |
AT1 | Ang II type 1 |
AT2 | Ang II type 2 |
ATPase | adenosine triphosphatase |
AT1R | angiotensin II type 1 receptor |
AT2R | angiotensin II type 2 receptor |
C21 | compound 21 |
CD | collecting duct |
CKD | chronic kidney disease |
COVID-19 | coronavirus disease 2019 |
COX | cyclooxygenase |
CREB | cAMP-responsive element-binding protein |
DDP | dipeptidyl aminopeptidase III |
DKD | diabetic kidney disease |
eGFR | estimated glomerular filtration rate |
ENaC | epithelial Na+ channel |
EP | specific PGE2 receptor |
ERK1/2 | extracellular signal-regulated kinase 1/2 |
Foxd1 | forkhead box protein D1 |
GFR | glomerular filtration rate |
HCO3− | bicarbonate |
IM | inner medulla |
JG | juxtaglomerular |
KO | knockout |
L-NAME | NG-nitro-L-arginine methyl ester |
MAPK | mitogen-activated protein kinase |
NEP | neutral endopeptidase/neprilysin |
NF-κB | nuclear factor-kappa B |
NHE3 | Na+/H+ exchanger 3 |
NO | nitric oxide |
NSAID | nonsteroidal anti-inflammatory drug |
PGE2 | prostaglandin E2 |
PGI2 | prostaglandin I2 |
POP | prolyloligopeptidase |
PRCP | prolylcarboxypeptidase |
(P)RR | (pro)renin receptor |
RAS | renin-angiotensin system |
RMIC | renomedullary interstitial cell |
SARS-CoV-2 | severe acute respiratory syndrome coronavirus 2 |
SGLT2 | sodium-glucose cotransporter-2 |
SHR | spontaneously hypertensive rat |
siRNA | small interfering RNA |
s(P)RR | soluble (pro)renin receptor |
STZ | streptozotocin |
TGFβ1 | transforming growth factor β1 |
VSMC | vascular smooth muscle cell. |
Authorship Contributions
Participated in research design: Lin, Geurts, Hassler, Batlle, Mirabito Colafella, Denton, Zhuo, Li, Ramkumar, Koizumi, Matsusaka, Nishiyama, Hoogduijn, Hoorn, Danser.
Wrote or contributed to the writing of the manuscript: Lin, Geurts, Hassler, Batlle, Mirabito Colafella, Denton, Zhuo, Li, Ramkumar, Koizumi, Matsusaka, Nishiyama, Hoogduijn, Hoorn, Danser.
Footnotes
This work was supported by Grants-in-Aid for Scientific Research from the Ministry of Education, Science, and Culture of Japan [Grant 18H03191] (to A.N.), National Institutes of Health National Institute of Diabetes and Digestive and Kidney Diseases [Grant 2R01DK067299-10A1], [Grant 2R01DK102429-03A1], and [Grant 1R01DK123144-01] (to X.C.L. and J.L.Z.), grants from the American Diabetes Association and the Pittsburgh Center for Kidney Research (to N.R.), National Health and Medical Research Council of Australia [Fellowship GNT1112125] (to K.M.M.C.) and [Fellowship GNT1136813] (to K.M.D.), National Institutes of Health National Institute of Allergy and Infectious Diseases [Grant 1R21AI166940-01] (to D.B.), a gift from the Joseph and Bessie Feinberg Foundation (to D.B.), and the Novo Nordisk Foundation [Grant 9013209] (to E.J.H.).
A.N. has received consulting fees, speaking honoraria, or both from Daiichi-Sankyo, Taisho, AstraZeneca, Tanabe-Mitsubishi, and Kowa. A.N. received research funds from Boehringer-Ingelheim, Daiichi-Sankyo, Taisho, and Bayer. A.H.J.D. received research funds from Alnylam Pharmaceuticals. D.B. is coinventor of patents entitled “Active low molecular weight variants of angiotensin converting enzyme 2,” “Active low molecular weight variants of angiotensin converting enzyme 2 (ACE2) for the treatment of diseases and conditions of the eye,” and “Shorter soluble forms of angiotensin converting enzyme 2 (ACE2) for treating and preventing coronavirus infection.” D.B. is founder of Angiotensin Therapeutics Inc. D.B. has received consulting fees from AstraZeneca, Relypsa, and Tricida, all unrelated to this work.
References
- Advani AKelly DJCox AJWhite KEAdvani SLThai KConnelly KAYuen DTrogadis JHerzenberg AM, et al. (2009) The (pro)renin receptor: site-specific and functional linkage to the vacuolar H+-ATPase in the kidney. Hypertension 54:261–269.PubMed [Abstract] [Google Scholar]
- Aguilera G, Kapur S, Feuillan P, Sunar-Akbasak B, Bathia AJ (1994) Developmental changes in angiotensin II receptor subtypes and AT1 receptor mRNA in rat kidney. Kidney Int 46:973–979. [Abstract] [Google Scholar]
- Alawi LF, Dhakal S, Emberesh SE, Sawant H, Hosawi A, Thanekar U, Grobe N, Elased KM (2021) Effects of angiotensin II type 1A receptor on ACE2, neprilysin and KIM-1 in two kidney one clip (2K1C) model of renovascular hypertension. Front Pharmacol 11:602985. [Europe PMC free article] [Abstract] [Google Scholar]
- Alawi LF, Emberesh SE, Owuor BA, Chodavarapu H, Fadnavis R, El-Amouri SS, Elased KM (2020) Effect of hyperglycemia and rosiglitazone on renal and urinary neprilysin in db/db diabetic mice. Physiol Rep 8:e14364. [Europe PMC free article] [Abstract] [Google Scholar]
- Alenina N, Xu P, Rentzsch B, Patkin EL, Bader M (2008) Genetically altered animal models for Mas and angiotensin-(1-7). Exp Physiol 93:528–537. [Abstract] [Google Scholar]
- Alexiou T, Boon WM, Denton DA, Nicolantonio RD, Walker LL, McKinley MJ, Campbell DJ (2005) Angiotensinogen and angiotensin-converting enzyme gene copy number and angiotensin and bradykinin peptide levels in mice. J Hypertens 23:945–954. [Abstract] [Google Scholar]
- Alge JL, Karakala N, Neely BA, Janech MG, Tumlin JA, Chawla LS, Shaw AD, Arthur JM; SAKInet Investigators (2013a) Urinary angiotensinogen and risk of severe AKI. Clin J Am Soc Nephrol 8:184–193. [Europe PMC free article] [Abstract] [Google Scholar]
- Alge JL, Karakala N, Neely BA, Janech MG, Velez JC, Arthur JM; SAKInet Investigators (2013b) Urinary angiotensinogen predicts adverse outcomes among acute kidney injury patients in the intensive care unit. Crit Care 17:R69. [Europe PMC free article] [Abstract] [Google Scholar]
- Ali Q, Wu Y, Hussain T (2013) Chronic AT2 receptor activation increases renal ACE2 activity, attenuates AT1 receptor function and blood pressure in obese Zucker rats. Kidney Int 84:931–939. [Europe PMC free article] [Abstract] [Google Scholar]
- Amin NB, Wang X, Mitchell JR, Lee DS, Nucci G, Rusnak JM (2015) Blood pressure-lowering effect of the sodium glucose co-transporter-2 inhibitor ertugliflozin, assessed via ambulatory blood pressure monitoring in patients with type 2 diabetes and hypertension. Diabetes Obes Metab 17:805–808. [Abstract] [Google Scholar]
- Ando S, Rahman MA, Butler GC, Senn BL, Floras JS (1995) Comparison of candoxatril and atrial natriuretic factor in healthy men. Effects on hemodynamics, sympathetic activity, heart rate variability, and endothelin. Hypertension 26:1160–1166. [Abstract] [Google Scholar]
- Ansary TM, Fujisawa Y, Rahman A, Nakano D, Hitomi H, Kobara H, Masaki T, Titze JM, Kitada K, Nishiyama A (2017) Responses of renal hemodynamics and tubular functions to acute sodium-glucose cotransporter 2 inhibitor administration in non-diabetic anesthetized rats. Sci Rep 7:9555. [Europe PMC free article] [Abstract] [Google Scholar]
- Antonucci R, Zaffanello M, Puxeddu E, Porcella A, Cuzzolin L, Pilloni MD, Fanos V (2012) Use of non-steroidal anti-inflammatory drugs in pregnancy: impact on the fetus and newborn. Curr Drug Metab 13:474–490. [Abstract] [Google Scholar]
- Arendse LB, Danser AHJ, Poglitsch M, Touyz RM, Burnett JC Jr, Llorens-Cortes C, Ehlers MR, Sturrock ED (2019) Novel therapeutic approaches targeting the renin-angiotensin system and associated peptides in hypertension and heart failure. Pharmacol Rev 71:539–570. [Europe PMC free article] [Abstract] [Google Scholar]
- Ba Aqeel SYe MWysocki JSanchez AKhattab ALores ERademaker AGao XBebu INelson RG, et al. ; Diabetes Control, Complications Trial (DCCT)/Epidemiology of Diabetes Interventions, Complications (EDIC) Study (2019) Urinary angiotensinogen antedates the development of stage 3 CKD in patients with type 1 diabetes mellitus. Physiol Rep 7:e14242. [Europe PMC free article] [Abstract] [Google Scholar]
- Bae EHFang FWilliams VRKonvalinka AZhou XPatel VBSong XJohn ROudit GYPei Y, et al. (2017) Murine recombinant angiotensin-converting enzyme 2 attenuates kidney injury in experimental Alport syndrome. Kidney Int 91:1347–1361. [Abstract] [Google Scholar]
- Balcarek J, Sevá Pessôa B, Bryson C, Azizi M, Ménard J, Garrelds IM, McGeehan G, Reeves RA, Griffith SG, Danser AH, et al. (2014) Multiple ascending dose study with the new renin inhibitor VTP-27999: nephrocentric consequences of too much renin inhibition. Hypertension 63:942–950. [Abstract] [Google Scholar]
- Barsha G, Mirabito Colafella KM, Walton SL, Gaspari TA, Spizzo I, Pinar AA, Hilliard Krause LM, Widdop RE, Samuel CS, Denton KM (2021) In aged females, the enhanced pressor response to angiotensin II is attenuated by estrogen replacement via an angiotensin type 2 receptor-mediated mechanism. Hypertension 78:128–137. [Abstract] [Google Scholar]
- Batenburg WW, Krop M, Garrelds IM, de Vries R, de Bruin RJ, Burcklé CA, Müller DN, Bader M, Nguyen G, Danser AH (2007) Prorenin is the endogenous agonist of the (pro)renin receptor. Binding kinetics of renin and prorenin in rat vascular smooth muscle cells overexpressing the human (pro)renin receptor. J Hypertens 25:2441–2453. [Abstract] [Google Scholar]
- Batenburg WW, Lu X, Leijten F, Maschke U, Müller DN, Danser AH (2011) Renin- and prorenin-induced effects in rat vascular smooth muscle cells overexpressing the human (pro)renin receptor: does (pro)renin-(pro)renin receptor interaction actually occur? Hypertension 58:1111–1119. [Abstract] [Google Scholar]
- Batlle D, Soler MJ, Sparks MA, Hiremath S, South AM, Welling PA, Swaminathan S; COVID-19 and ACE2 in Cardiovascular, Lung, and Kidney Working Group (2020a) Acute kidney injury in COVID-19: emerging evidence of a distinct pathophysiology. J Am Soc Nephrol 31:1380–1383. [Europe PMC free article] [Abstract] [Google Scholar]
- Batlle D, Wysocki J, Satchell K (2020b) Soluble angiotensin-converting enzyme 2: a potential approach for coronavirus infection therapy? Clin Sci (Lond) 134:543–545. [Abstract] [Google Scholar]
- Bernstein KE, Ong FS, Blackwell WL, Shah KH, Giani JF, Gonzalez-Villalobos RA, Shen XZ, Fuchs S, Touyz RM (2012) A modern understanding of the traditional and nontraditional biological functions of angiotensin-converting enzyme. Pharmacol Rev 65:1–46. [Europe PMC free article] [Abstract] [Google Scholar]
- Bhala NEmberson JMerhi AAbramson SArber NBaron JABombardier CCannon CFarkouh MEFitzGerald GA, et al. ; Coxib and traditional NSAID Trialists’ (CNT) Collaboration (2013) Vascular and upper gastrointestinal effects of non-steroidal anti-inflammatory drugs: meta-analyses of individual participant data from randomised trials. Lancet 382:769–779. [Europe PMC free article] [Abstract] [Google Scholar]
- Blanchard AVargas-Poussou RVallet MCaumont-Prim AAllard JDesport EDubourg LMonge MBergerot DBaron S, et al. (2015) Indomethacin, amiloride, or eplerenone for treating hypokalemia in Gitelman syndrome. J Am Soc Nephrol 26:468–475. [Europe PMC free article] [Abstract] [Google Scholar]
- Boerrigter G, Soergel DG, Violin JD, Lark MW, Burnett JC Jr (2012) TRV120027, a novel β-arrestin biased ligand at the angiotensin II type I receptor, unloads the heart and maintains renal function when added to furosemide in experimental heart failure. Circ Heart Fail 5:627–634. [Abstract] [Google Scholar]
- Boorsma EM, Beusekamp JC, Ter Maaten JM, Figarska SM, Danser AHJ, van Veldhuisen DJ, van der Meer P, Heerspink HJL, Damman K, Voors AA (2021) Effects of empagliflozin on renal sodium and glucose handling in patients with acute heart failure. Eur J Heart Fail 23:68–78. [Europe PMC free article] [Abstract] [Google Scholar]
- Bosnyak S, Welungoda IK, Hallberg A, Alterman M, Widdop RE, Jones ES (2010) Stimulation of angiotensin AT2 receptors by the non-peptide agonist, Compound 21, evokes vasodepressor effects in conscious spontaneously hypertensive rats. Br J Pharmacol 159:709–716. [Europe PMC free article] [Abstract] [Google Scholar]
- Bosnyak S, Widdop RE, Denton KM, Jones ES (2012) Differential mechanisms of ang (1-7)-mediated vasodepressor effect in adult and aged candesartan-treated rats. Int J Hypertens 2012:192567. [Europe PMC free article] [Abstract] [Google Scholar]
- Bovée DMRen LUijl EClahsen-van Groningen MCvan Veghel RGarrelds IMDomenig OPoglitsch MZlatev IKim JB, et al. (2021) Renoprotective effects of small interfering RNA targeting liver angiotensinogen in experimental chronic kidney disease. Hypertension 77:1600–1612. [Abstract] [Google Scholar]
- Brown CK, Madauss K, Lian W, Beck MR, Tolbert WD, Rodgers DW (2001) Structure of neurolysin reveals a deep channel that limits substrate access. Proc Natl Acad Sci USA 98:3127–3132. [Europe PMC free article] [Abstract] [Google Scholar]
- Brown RD, Hilliard LM, Head GA, Jones ES, Widdop RE, Denton KM (2012) Sex differences in the pressor and tubuloglomerular feedback response to angiotensin II. Hypertension 59:129–135. [Abstract] [Google Scholar]
- Brunskill EW, Sequeira-Lopez ML, Pentz ES, Lin E, Yu J, Aronow BJ, Potter SS, Gomez RA (2011) Genes that confer the identity of the renin cell. J Am Soc Nephrol 22:2213–2225. [Europe PMC free article] [Abstract] [Google Scholar]
- Burcklé C, Bader M (2006) Prorenin and its ancient receptor. Hypertension 48:549–551. [Abstract] [Google Scholar]
- Campbell DJ (1996) Endogenous angiotensin II levels and the mechanism of action of angiotensin-converting enzyme inhibitors and angiotensin receptor type 1 antagonists. Clin Exp Pharmacol Physiol 23 (Suppl 3):S125–S131. [Abstract] [Google Scholar]
- Campbell DJ, Duncan AM, Kladis A, Harrap SB (1995a) Angiotensin peptides in spontaneously hypertensive and normotensive Donryu rats. Hypertension 25:928–934. [Abstract] [Google Scholar]
- Campbell DJ, Kladis A, Valentijn AJ (1995b) Effects of losartan on angiotensin and bradykinin peptides and angiotensin-converting enzyme. J Cardiovasc Pharmacol 26:233–240. [Abstract] [Google Scholar]
- Campbell DJ, Lawrence AC, Towrie A, Kladis A, Valentijn AJ (1991) Differential regulation of angiotensin peptide levels in plasma and kidney of the rat. Hypertension 18:763–773. [Abstract] [Google Scholar]
- Campbell DJ, Nussberger J, Stowasser M, Danser AH, Morganti A, Frandsen E, Ménard J (2009) Activity assays and immunoassays for plasma renin and prorenin: information provided and precautions necessary for accurate measurement. Clin Chem 55:867–877. [Abstract] [Google Scholar]
- Campbell DJ, Rong P, Kladis A, Rees B, Ganten D, Skinner SL (1995c) Angiotensin and bradykinin peptides in the TGR(mRen-2)27 rat. Hypertension 25:1014–1020. [Abstract] [Google Scholar]
- Campbell DJ, Zhang Y, Kelly DJ, Gilbert RE, McCarthy DJ, Shi W, Smyth GK (2011) Aliskiren increases bradykinin and tissue kallikrein mRNA levels in the heart. Clin Exp Pharmacol Physiol 38:623–631. [Abstract] [Google Scholar]
- Cao W, Jin L, Zhou Z, Yang M, Wu C, Wu L, Cui S (2016) Overexpression of intrarenal renin-angiotensin system in human acute tubular necrosis. Kidney Blood Press Res 41:746–756. [Abstract] [Google Scholar]
- Carneiro de Morais CP, Polidoro JZ, Ralph DL, Pessoa TD, Oliveira-Souza M, Barauna VG, Rebouças NA, Malnic G, McDonough AA, Girardi AC (2015) Proximal tubule NHE3 activity is inhibited by beta-arrestin-biased angiotensin II type 1 receptor signaling. Am J Physiol Cell Physiol 309:C541–C550. [Europe PMC free article] [Abstract] [Google Scholar]
- Castellanos-Rivera RM, Pentz ES, Lin E, Gross KW, Medrano S, Yu J, Sequeira-Lopez ML, Gomez RA (2015) Recombination signal binding protein for Ig-κJ region regulates juxtaglomerular cell phenotype by activating the myo-endocrine program and suppressing ectopic gene expression. J Am Soc Nephrol 26:67–80. [Europe PMC free article] [Abstract] [Google Scholar]
- Castellanos Rivera RM, Monteagudo MC, Pentz ES, Glenn ST, Gross KW, Carretero O, Sequeira-Lopez ML, Gomez RA (2011) Transcriptional regulator RBP-J regulates the number and plasticity of renin cells. Physiol Genomics 43:1021–1028. [Europe PMC free article] [Abstract] [Google Scholar]
- Castelo-Branco RC, Leite-Delova DC, de Mello-Aires M (2013) Dose-dependent effects of angiotensin-(1-7) on the NHE3 exchanger and [Ca(2+)](i) in in vivo proximal tubules. Am J Physiol Renal Physiol 304:F1258–F1265. [Europe PMC free article] [Abstract] [Google Scholar]
- Catella-Lawson F, McAdam B, Morrison BW, Kapoor S, Kujubu D, Antes L, Lasseter KC, Quan H, Gertz BJ, FitzGerald GA (1999) Effects of specific inhibition of cyclooxygenase-2 on sodium balance, hemodynamics, and vasoactive eicosanoids. J Pharmacol Exp Ther 289:735–741. [Abstract] [Google Scholar]
- Cerniello FM, Carretero OA, Longo Carbajosa NA, Cerrato BD, Santos RA, Grecco HE, Gironacci MM (2017) MAS1 receptor trafficking involves ERK1/2 activation through a β-arrestin2-dependent pathway. Hypertension 70:982–989. [Europe PMC free article] [Abstract] [Google Scholar]
- Chappell MC (2012) Nonclassical renin-angiotensin system and renal function. Compr Physiol 2:2733–2752. [Europe PMC free article] [Abstract] [Google Scholar]
- Chappell MC, Pirro NT, South AM, Gwathmey TM (2021) Concerns on the specificity of commercial ELISAs for the measurement of angiotensin (1-7) and angiotensin II in human plasma. Hypertension 77:e29–e31. [Europe PMC free article] [Abstract] [Google Scholar]
- Chappellaz ML, Smith FG (2007) Systemic and renal hemodynamic effects of the AT1 receptor antagonist, ZD 7155, and the AT2 receptor antagonist, PD 123319, in conscious lambs. Pflugers Arch 453:477–486. [Abstract] [Google Scholar]
- Chen L, Chou CL, Knepper MA (2021) A comprehensive map of mRNAs and their isoforms across all 14 renal tubule segments of mouse. J Am Soc Nephrol 32:897–912. [Europe PMC free article] [Abstract] [Google Scholar]
- Chen X, Li W, Yoshida H, Tsuchida S, Nishimura H, Takemoto F, Okubo S, Fogo A, Matsusaka T, Ichikawa I (1997) Targeting deletion of angiotensin type 1B receptor gene in the mouse. Am J Physiol 272:F299–F304. [Abstract] [Google Scholar]
- Cheng HF, Wang JL, Zhang MZ, Miyazaki Y, Ichikawa I, McKanna JA, Harris RC (1999) Angiotensin II attenuates renal cortical cyclooxygenase-2 expression. J Clin Invest 103:953–961. [Europe PMC free article] [Abstract] [Google Scholar]
- Cheng HF, Wang SW, Zhang MZ, McKanna JA, Breyer R, Harris RC (2002) Prostaglandins that increase renin production in response to ACE inhibition are not derived from cyclooxygenase-1. Am J Physiol Regul Integr Comp Physiol 283:R638–R646. [Abstract] [Google Scholar]
- Cherney DZPerkins BASoleymanlou NMaione MLai VLee AFagan NMWoerle HJJohansen OEBroedl UC, et al. (2014) Renal hemodynamic effect of sodium-glucose cotransporter 2 inhibition in patients with type 1 diabetes mellitus. Circulation 129:587–597. [Abstract] [Google Scholar]
- Cherney DZIDekkers CCJBarbour SJCattran DAbdul Gafor AHGreasley PJLaverman GDLim SKDi Tanna GLReich HN, et al. ; DIAMOND investigators (2020) Effects of the SGLT2 inhibitor dapagliflozin on proteinuria in non-diabetic patients with chronic kidney disease (DIAMOND): a randomised, double-blind, crossover trial. Lancet Diabetes Endocrinol 8:582–593. [Abstract] [Google Scholar]
- Cook JL, Zhang Z, Re RN (2001) In vitro evidence for an intracellular site of angiotensin action. Circ Res 89:1138–1146. [Abstract] [Google Scholar]
- Cousin C, Bracquart D, Contrepas A, Corvol P, Muller L, Nguyen G (2009) Soluble form of the (pro)renin receptor generated by intracellular cleavage by furin is secreted in plasma. Hypertension 53:1077–1082. [Abstract] [Google Scholar]
- Crackower MASarao ROudit GYYagil CKozieradzki IScanga SEOliveira-dos-Santos AJda Costa JZhang LPei Y, et al. (2002) Angiotensin-converting enzyme 2 is an essential regulator of heart function. Nature 417:822–828. [Abstract] [Google Scholar]
- Crowley SD, Gurley SB, Herrera MJ, Ruiz P, Griffiths R, Kumar AP, Kim HS, Smithies O, Le TH, Coffman TM (2006) Angiotensin II causes hypertension and cardiac hypertrophy through its receptors in the kidney. Proc Natl Acad Sci USA 103:17985–17990. [Europe PMC free article] [Abstract] [Google Scholar]
- Crowley SDGurley SBOliverio MIPazmino AKGriffiths RFlannery PJSpurney RFKim HSSmithies OLe TH, et al. (2005) Distinct roles for the kidney and systemic tissues in blood pressure regulation by the renin-angiotensin system. J Clin Invest 115:1092–1099. [Europe PMC free article] [Abstract] [Google Scholar]
- Crowley SD, Rudemiller NP (2017) Immunologic effects of the renin-angiotensin system. J Am Soc Nephrol 28:1350–1361. [Europe PMC free article] [Abstract] [Google Scholar]
- Cruciat CM, Ohkawara B, Acebron SP, Karaulanov E, Reinhard C, Ingelfinger D, Boutros M, Niehrs C (2010) Requirement of prorenin receptor and vacuolar H+-ATPase-mediated acidification for Wnt signaling. Science 327:459–463. [Abstract] [Google Scholar]
- Czerniecki SMCruz NMHarder JLMenon RAnnis JOtto EAGulieva REIslas LVKim YKTran LM, et al. (2018) High-throughput screening enhances kidney organoid differentiation from human pluripotent stem cells and enables automated multidimensional phenotyping. Cell Stem Cell 22:929–940.e4. [Europe PMC free article] [Abstract] [Google Scholar]
- Dadam FM, Cisternas CD, Macchione AF, Godino A, Antunes-Rodrigues J, Cambiasso MJ, Vivas LM, Caeiro XE (2017) Sex chromosome complement involvement in angiotensin receptor sexual dimorphism. Mol Cell Endocrinol 447:98–105. [Abstract] [Google Scholar]
- Damman KGori MClaggett BJhund PSSenni MLefkowitz MPPrescott MFShi VCRouleau JLSwedberg K, et al. (2018) Renal effects and associated outcomes during angiotensin-neprilysin inhibition in heart failure. JACC Heart Fail 6:489–498. [Abstract] [Google Scholar]
- Danser AH (2007) Novel drugs targeting hypertension: renin inhibitors. J Cardiovasc Pharmacol 50:105–111. [Abstract] [Google Scholar]
- Danser AH, Deinum J (2005) Renin, prorenin and the putative (pro)renin receptor. Hypertension 46:1069–1076. [Abstract] [Google Scholar]
- Danser AH, Derkx FH, Schalekamp MA, Hense HW, Riegger GA, Schunkert H (1998) Determinants of interindividual variation of renin and prorenin concentrations: evidence for a sexual dimorphism of (pro)renin levels in humans. J Hypertens 16:853–862. [Abstract] [Google Scholar]
- Danser AH, Koning MM, Admiraal PJ, Derkx FH, Verdouw PD, Schalekamp MA (1992) Metabolism of angiotensin I by different tissues in the intact animal. Am J Physiol 263:H418–H428. [Abstract] [Google Scholar]
- Danser AH, van den Dorpel MA, Deinum J, Derkx FH, Franken AA, Peperkamp E, de Jong PT, Schalekamp MA (1989) Renin, prorenin, and immunoreactive renin in vitreous fluid from eyes with and without diabetic retinopathy. J Clin Endocrinol Metab 68:160–167. [Abstract] [Google Scholar]
- Danser AH, van den Meiracker AH (2015) Heart failure: new data do not SUPPORT triple RAAS blockade. Nat Rev Nephrol 11:260–262. [Abstract] [Google Scholar]
- Danser AHJ, Epstein M, Batlle D (2020) Renin-angiotensin system blockers and the COVID-19 pandemic: at present there is no evidence to abandon renin-angiotensin system blockers. Hypertension 75:1382–1385. [Europe PMC free article] [Abstract] [Google Scholar]
- Danyel LA, Schmerler P, Paulis L, Unger T, Steckelings UM (2013) Impact of AT2-receptor stimulation on vascular biology, kidney function, and blood pressure. Integr Blood Press Control 6:153–161. [Europe PMC free article] [Abstract] [Google Scholar]
- Daryadel ABourgeois SFigueiredo MFGomes Moreira AKampik NBOberli LMohebbi NLu XMeima MEDanser AH, et al. (2016) Colocalization of the (pro)renin receptor/Atp6ap2 with H+-ATPases in mouse kidney but prorenin does not acutely regulate intercalated cell H+-ATPase activity. PLoS One 11:e0147831. [Europe PMC free article] [Abstract] [Google Scholar]
- Davisson RL, Ding Y, Stec DE, Catterall JF, Sigmund CD (1999) Novel mechanism of hypertension revealed by cell-specific targeting of human angiotensinogen in transgenic mice. Physiol Genomics 1:3–9. [Abstract] [Google Scholar]
- de Lannoy LM, Danser AH, van Kats JP, Schoemaker RG, Saxena PR, Schalekamp MA (1997) Renin-angiotensin system components in the interstitial fluid of the isolated perfused rat heart. Local production of angiotensin I. Hypertension 29:1240–1251. [Abstract] [Google Scholar]
- De Mello WC (1998) Intracellular angiotensin II regulates the inward calcium current in cardiac myocytes. Hypertension 32:976–982. [Abstract] [Google Scholar]
- Del Prete DGambaro GLupo AAnglani FBrezzi BMagistroni RGraziotto RFurci LModena FBernich P, et al. (2003) Precocious activation of genes of the renin-angiotensin system and the fibrogenic cascade in IgA glomerulonephritis. Kidney Int 64:149–159. [Abstract] [Google Scholar]
- DelliPizzi AM, Hilchey SD, Bell-Quilley CP (1994) Natriuretic action of angiotensin(1-7). Br J Pharmacol 111:1–3. [Europe PMC free article] [Abstract] [Google Scholar]
- Dibo P, Marañón RO, Chandrashekar K, Mazzuferi F, Silva GB, Juncos LA, Juncos LI (2019) Angiotensin-(1-7) inhibits sodium transport via Mas receptor by increasing nitric oxide production in thick ascending limb. Physiol Rep 7:e14015. [Europe PMC free article] [Abstract] [Google Scholar]
- Dinchuk JECar BDFocht RJJohnston JJJaffee BDCovington MBContel NREng VMCollins RJCzerniak PM, et al. (1995) Renal abnormalities and an altered inflammatory response in mice lacking cyclooxygenase II. Nature 378:406–409. [Abstract] [Google Scholar]
- Domenig OManzel AGrobe NKönigshausen EKaltenecker CCKovarik JJStegbauer JGurley SBvan Oyen DAntlanger M, et al. (2016) Neprilysin is a mediator of alternative renin-angiotensin-system activation in the murine and human kidney. Sci Rep 6:33678. [Europe PMC free article] [Abstract] [Google Scholar]
- Donoghue MHsieh FBaronas EGodbout KGosselin MStagliano NDonovan MWoolf BRobison KJeyaseelan R, et al. (2000) A novel angiotensin-converting enzyme-related carboxypeptidase (ACE2) converts angiotensin I to angiotensin 1-9. Circ Res 87:E1–E9. [Abstract] [Google Scholar]
- Eggena P, Zhu JH, Sereevinyayut S, Giordani M, Clegg K, Andersen PC, Hyun P, Barrett JD (1996) Hepatic angiotensin II nuclear receptors and transcription of growth-related factors. J Hypertens 14:961–968. [Abstract] [Google Scholar]
- Eickhoff MKDekkers CCJKramers BJLaverman GDFrimodt-Møller MJørgensen NRFaber JDanser AHJGansevoort RTRossing P, et al. (2019) Effects of dapagliflozin on volume status when added to renin-angiotensin system inhibitors. J Clin Med 8:779. [Europe PMC free article] [Abstract] [Google Scholar]
- Esteban VHeringer-Walther SSterner-Kock Ade Bruin Rvan den Engel SWang YMezzano SEgido JSchultheiss HPRuiz-Ortega M, et al. (2009) Angiotensin-(1-7) and the g protein-coupled receptor MAS are key players in renal inflammation. PLoS One 4:e5406. [Europe PMC free article] [Abstract] [Google Scholar]
- Fang FLiu GCZhou XYang SReich HNWilliams VHu APan JKonvalinka AOudit GY, et al. (2013) Loss of ACE2 exacerbates murine renal ischemia-reperfusion injury. PLoS One 8:e71433. [Europe PMC free article] [Abstract] [Google Scholar]
- Fang HXu CLu AZou CJXie SChen YZhou LLiu MWang LWang W, et al. (2017) (Pro)renin receptor mediates albumin-induced cellular responses: role of site-1 protease-derived soluble (pro)renin receptor in renal epithelial cells. Am J Physiol Cell Physiol 313:C632–C643. [Europe PMC free article] [Abstract] [Google Scholar]
- Feldt S, Maschke U, Dechend R, Luft FC, Muller DN (2008) The putative (pro)renin receptor blocker HRP fails to prevent (pro)renin signaling. J Am Soc Nephrol 19:743–748. [Europe PMC free article] [Abstract] [Google Scholar]
- Feng Y, Peng K, Luo R, Wang F, Yang T (2021) Site-1 protease-derived soluble (pro)renin receptor contributes to angiotensin II-induced hypertension in mice. Hypertension 77:405–416. [Europe PMC free article] [Abstract] [Google Scholar]
- Ferrão FM, Cardoso LHD, Drummond HA, Li XC, Zhuo JL, Gomes DS, Lara LS, Vieyra A, Lowe J (2017) Luminal ANG II is internalized as a complex with AT1R/AT2R heterodimers to target endoplasmic reticulum in LLC-PK1 cells. Am J Physiol Renal Physiol 313:F440–F449. [Europe PMC free article] [Abstract] [Google Scholar]
- Fisher ND, Danser AH, Nussberger J, Dole WP, Hollenberg NK (2008) Renal and hormonal responses to direct renin inhibition with aliskiren in healthy humans. Circulation 117:3199–3205. [Abstract] [Google Scholar]
- Forbes TAHowden SELawlor KPhipson BMaksimovic JHale LWilson SQuinlan CHo GHolman K, et al. (2018) Patient-iPSC-derived kidney organoids show functional validation of a ciliopathic renal phenotype and reveal underlying pathogenetic mechanisms. Am J Hum Genet 102:816–831. [Europe PMC free article] [Abstract] [Google Scholar]
- Forrester SJ, Booz GW, Sigmund CD, Coffman TM, Kawai T, Rizzo V, Scalia R, Eguchi S (2018) Angiotensin II signal transduction: an update on mechanisms of physiology and pathophysiology. Physiol Rev 98:1627–1738. [Europe PMC free article] [Abstract] [Google Scholar]
- Freedman BSBrooks CRLam AQFu HMorizane RAgrawal VSaad AFLi MKHughes MRWerff RV, et al. (2015) Modelling kidney disease with CRISPR-mutant kidney organoids derived from human pluripotent epiblast spheroids. Nat Commun 6:8715. [Europe PMC free article] [Abstract] [Google Scholar]
- Fried LFEmanuele NZhang JHBrophy MConner TADuckworth WLeehey DJMcCullough PAO’Connor TPalevsky PM, et al. ; VA NEPHRON-D Investigators (2013) Combined angiotensin inhibition for the treatment of diabetic nephropathy. N Engl J Med 369:1892–1903. [Abstract] [Google Scholar]
- Fu ZWang FLiu XHu JSu JLu XLu ACho JMSymons JDZou CJ, et al. (2021) Soluble (pro)renin receptor induces endothelial dysfunction and hypertension in mice with diet-induced obesity via activation of angiotensin II type 1 receptor. Clin Sci (Lond) 135:793–810. [Europe PMC free article] [Abstract] [Google Scholar]
- Fukushima AKinugawa SHomma TMasaki YFurihata TAbe TSuga TTakada SKadoguchi TOkita K, et al. (2013) Increased plasma soluble (pro)renin receptor levels are correlated with renal dysfunction in patients with heart failure. Int J Cardiol 168:4313–4314. [Abstract] [Google Scholar]
- Gaidarov I, Adams J, Frazer J, Anthony T, Chen X, Gatlin J, Semple G, Unett DJ (2018) Angiotensin (1-7) does not interact directly with MAS1, but can potently antagonize signaling from the AT1 receptor. Cell Signal 50:9–24. [Abstract] [Google Scholar]
- Galandrin SDenis CBoularan CMarie JM’Kadmi CPilette CDubroca CNicaise YSeguelas MHN’Guyen D, et al. (2016) Cardioprotective angiotensin-(1-7) peptide acts as a natural-biased ligand at the angiotensin II type 1 receptor. Hypertension 68:1365–1374. [Abstract] [Google Scholar]
- Garcia NH, Garvin JL (1994) Angiotensin 1-7 has a biphasic effect on fluid absorption in the proximal straight tubule. J Am Soc Nephrol 5:1133–1138. [Abstract] [Google Scholar]
- Garreta EPrado PTarantino COria RFanlo LMartí EZalvidea DTrepat XRoca-Cusachs PGavaldà-Navarro A, et al. (2019) Fine tuning the extracellular environment accelerates the derivation of kidney organoids from human pluripotent stem cells. Nat Mater 18:397–405. [Europe PMC free article] [Abstract] [Google Scholar]
- Gasc JM, Shanmugam S, Sibony M, Corvol P (1994) Tissue-specific expression of type 1 angiotensin II receptor subtypes. An in situ hybridization study. Hypertension 24:531–537. [Abstract] [Google Scholar]
- Gasparo M, Catt KJ, Inagami T, Wright JW, Unger T (2000) International union of pharmacology. XXIII. The angiotensin II receptors. Pharmacol Rev 52:415.–. [Abstract] [Google Scholar]
- Gava E, Samad-Zadeh A, Zimpelmann J, Bahramifarid N, Kitten GT, Santos RA, Touyz RM, Burns KD (2009) Angiotensin-(1-7) activates a tyrosine phosphatase and inhibits glucose-induced signalling in proximal tubular cells. Nephrol Dial Transplant 24:1766–1773. [Europe PMC free article] [Abstract] [Google Scholar]
- Giani JF, Eriguchi M, Bernstein EA, Katsumata M, Shen XZ, Li L, McDonough AA, Fuchs S, Bernstein KE, Gonzalez-Villalobos RA (2017) Renal tubular angiotensin converting enzyme is responsible for nitro-L-arginine methyl ester (L-NAME)-induced salt sensitivity. Kidney Int 91:856–867. [Europe PMC free article] [Abstract] [Google Scholar]
- Giani JFJanjulia TKamat NSeth DMBlackwell WLShah KHShen XZFuchs SDelpire EToblli JE, et al. (2014) Renal angiotensin-converting enzyme is essential for the hypertension induced by nitric oxide synthesis inhibition. J Am Soc Nephrol 25:2752–2763. [Europe PMC free article] [Abstract] [Google Scholar]
- Gibson RE, Thorpe HH, Cartwright ME, Frank JD, Schorn TW, Bunting PB, Siegl PK (1991) Angiotensin II receptor subtypes in renal cortex of rats and rhesus monkeys. Am J Physiol 261:F512–F518. [Abstract] [Google Scholar]
- Gilbert RE, Wu LL, Kelly DJ, Cox A, Wilkinson-Berka JL, Johnston CI, Cooper ME (1999) Pathological expression of renin and angiotensin II in the renal tubule after subtotal nephrectomy. Implications for the pathogenesis of tubulointerstitial fibrosis. Am J Pathol 155:429–440. [Europe PMC free article] [Abstract] [Google Scholar]
- Gironacci MM, Adamo HP, Corradi G, Santos RA, Ortiz P, Carretero OA (2011) Angiotensin (1-7) induces MAS receptor internalization. Hypertension 58:176–181. [Europe PMC free article] [Abstract] [Google Scholar]
- Gomez RA, Sequeira-Lopez MLS (2018) Renin cells in homeostasis, regeneration and immune defence mechanisms. Nat Rev Nephrol 14:231–245. [Europe PMC free article] [Abstract] [Google Scholar]
- Gonzalez AA, Green T, Luffman C, Bourgeois CR, Gabriel Navar L, Prieto MC (2014a) Renal medullary cyclooxygenase-2 and (pro)renin receptor expression during angiotensin II-dependent hypertension. Am J Physiol Renal Physiol 307:F962–F970. [Europe PMC free article] [Abstract] [Google Scholar]
- Gonzalez AA, Lara LS, Luffman C, Seth DM, Prieto MC (2011) Soluble form of the (pro)renin receptor is augmented in the collecting duct and urine of chronic angiotensin II-dependent hypertensive rats. Hypertension 57:859–864. [Europe PMC free article] [Abstract] [Google Scholar]
- Gonzalez AA, Luffman C, Bourgeois CR, Vio CP, Prieto MC (2013) Angiotensin II-independent upregulation of cyclooxygenase-2 by activation of the (Pro)renin receptor in rat renal inner medullary cells. Hypertension 61:443–449. [Europe PMC free article] [Abstract] [Google Scholar]
- Gonzalez AA, Womack JP, Liu L, Seth DM, Prieto MC (2014b) Angiotensin II increases the expression of (pro)renin receptor during low-salt conditions. Am J Med Sci 348:416–422. [Europe PMC free article] [Abstract] [Google Scholar]
- Gonzalez-Villalobos RA, Billet S, Kim C, Satou R, Fuchs S, Bernstein KE, Navar LG (2011) Intrarenal angiotensin-converting enzyme induces hypertension in response to angiotensin I infusion. J Am Soc Nephrol 22:449–459. [Europe PMC free article] [Abstract] [Google Scholar]
- Gonzalez-Villalobos RAJanjoulia TFletcher NKGiani JFNguyen MTRiquier-Brison ADSeth DMFuchs SEladari DPicard N, et al. (2013) The absence of intrarenal ACE protects against hypertension. J Clin Invest 123:2011–2023. [Europe PMC free article] [Abstract] [Google Scholar]
- Gonzalez-Villalobos RA, Seth DM, Satou R, Horton H, Ohashi N, Miyata K, Katsurada A, Tran DV, Kobori H, Navar LG (2008) Intrarenal angiotensin II and angiotensinogen augmentation in chronic angiotensin II-infused mice. Am J Physiol Renal Physiol 295:F772–F779. [Europe PMC free article] [Abstract] [Google Scholar]
- Grady EF, Sechi LA, Griffin CA, Schambelan M, Kalinyak JE (1991) Expression of AT2 receptors in the developing rat fetus. J Clin Invest 88:921–933. [Europe PMC free article] [Abstract] [Google Scholar]
- Green T, Rodriguez J, Navar LG (2010) Augmented cyclooxygenase-2 effects on renal function during varying states of angiotensin II. Am J Physiol Renal Physiol 299:F954–F962. [Europe PMC free article] [Abstract] [Google Scholar]
- Gribouval OGonzales MNeuhaus TAziza JBieth ELaurent NBouton JMFeuillet FMakni SBen Amar H, et al. (2005) Mutations in genes in the renin-angiotensin system are associated with autosomal recessive renal tubular dysgenesis. Nat Genet 37:964–968. [Abstract] [Google Scholar]
- Grobe N, Leiva O, Morris M, Elased KM (2015) Loss of prolyl carboxypeptidase in two-kidney, one-clip goldblatt hypertensive mice. PLoS One 10:e0117899. [Europe PMC free article] [Abstract] [Google Scholar]
- Grobe N, Weir NM, Leiva O, Ong FS, Bernstein KE, Schmaier AH, Morris M, Elased KM (2013) Identification of prolyl carboxypeptidase as an alternative enzyme for processing of renal angiotensin II using mass spectrometry. Am J Physiol Cell Physiol 304:C945–C953. [Europe PMC free article] [Abstract] [Google Scholar]
- Gröne HJ, Simon M, Fuchs E (1992) Autoradiographic characterization of angiotensin receptor subtypes in fetal and adult human kidney. Am J Physiol 262:F326–F331. [Abstract] [Google Scholar]
- Gross V, Schunck WH, Honeck H, Milia AF, Kärgel E, Walther T, Bader M, Inagami T, Schneider W, Luft FC (2000) Inhibition of pressure natriuresis in mice lacking the AT2 receptor. Kidney Int 57:191–202. [Abstract] [Google Scholar]
- Guessoum O, de Goes Martini A, Sequeira-Lopez MLS, Gomez RA (2021) Deciphering the identity of renin cells in health and disease. Trends Mol Med 27:280–292. [Europe PMC free article] [Abstract] [Google Scholar]
- Gurley SBAllred ALe THGriffiths RMao LPhilip NHaystead TADonoghue MBreitbart REActon SL, et al. (2006) Altered blood pressure responses and normal cardiac phenotype in ACE2-null mice. J Clin Invest 116:2218–2225. [Europe PMC free article] [Abstract] [Google Scholar]
- Gurley SBRiquier-Brison ADMSchnermann JSparks MAAllen AMHaase VHSnouwaert JNLe THMcDonough AAKoller BH, et al. (2011) AT1A angiotensin receptors in the renal proximal tubule regulate blood pressure. Cell Metab 13:469–475. [Europe PMC free article] [Abstract] [Google Scholar]
- Habibi J, Aroor AR, Das NA, Manrique-Acevedo CM, Johnson MS, Hayden MR, Nistala R, Wiedmeyer C, Chandrasekar B, DeMarco VG (2019) The combination of a neprilysin inhibitor (sacubitril) and angiotensin-II receptor blocker (valsartan) attenuates glomerular and tubular injury in the Zucker obese rat. Cardiovasc Diabetol 18:40. [Europe PMC free article] [Abstract] [Google Scholar]
- Haithcock D, Jiao H, Cui XL, Hopfer U, Douglas JG (1999) Renal proximal tubular AT2 receptor: signaling and transport. J Am Soc Nephrol 10 (Suppl 11):S69–S74. [Abstract] [Google Scholar]
- Haller HIto SIzzo JL JrJanuszewicz AKatayama SMenne JMimran ARabelink TJRitz ERuilope LM, et al. ; ROADMAP Trial Investigators (2011) Olmesartan for the delay or prevention of microalbuminuria in type 2 diabetes. N Engl J Med 364:907–917. [Abstract] [Google Scholar]
- Haller H, Lindschau C, Erdmann B, Quass P, Luft FC (1996) Effects of intracellular angiotensin II in vascular smooth muscle cells. Circ Res 79:765–772. [Abstract] [Google Scholar]
- Han E, Shin E, Kim G, Lee JY, Lee YH, Lee BW, Kang ES, Cha BS (2018) Combining SGLT2 inhibition with a thiazolidinedione additively attenuate the very early phase of diabetic nephropathy progression in type 2 diabetes mellitus. Front Endocrinol (Lausanne) 9:412. [Europe PMC free article] [Abstract] [Google Scholar]
- Harris RC, McKanna JA, Akai Y, Jacobson HR, Dubois RN, Breyer MD (1994) Cyclooxygenase-2 is associated with the macula densa of rat kidney and increases with salt restriction. J Clin Invest 94:2504–2510. [Europe PMC free article] [Abstract] [Google Scholar]
- Harris RC, Zhang MZ, Cheng HF (2004) Cyclooxygenase-2 and the renal renin-angiotensin system. Acta Physiol Scand 181:543–547. [Abstract] [Google Scholar]
- Harrison-Bernard LM, Navar LG, Ho MM, Vinson GP, el-Dahr SS (1997) Immunohistochemical localization of ANG II AT1 receptor in adult rat kidney using a monoclonal antibody. Am J Physiol 273:F170–F177. [Abstract] [Google Scholar]
- Hassler LWysocki JGelarden ISharma ITomatsidou AYe MGula HNicoleascu VRandall GPshenychnyi S, et al. (2022) A novel soluble ACE2 protein provides lung and kidney protection in mice susceptible to lethal SARS-CoV-2 infection. J Am Soc Nephrol ASN.2021091209 (in press). [Europe PMC free article] [Abstract] [Google Scholar]
- Haynes RJudge PKStaplin NHerrington WGStorey BCBethel ABowman LBrunskill NCockwell PHill M, et al. (2018) Effects of sacubitril/valsartan versus irbesartan in patients with chronic kidney disease. Circulation 138:1505–1514. [Abstract] [Google Scholar]
- Healy DP, Ye MQ, Troyanovskaya M (1995) Localization of angiotensin II type 1 receptor subtype mRNA in rat kidney. Am J Physiol 268:F220–F226. [Abstract] [Google Scholar]
- Heerspink HJLStefánsson BVCorrea-Rotter RChertow GMGreene THou FFMann JFEMcMurray JJVLindberg MRossing P, et al. ; DAPA-CKD Trial Committees and Investigators (2020) Dapagliflozin in patients with chronic kidney disease. N Engl J Med 383:1436–1446. [Abstract] [Google Scholar]
- Heitsch H, Brovkovych S, Malinski T, Wiemer G (2001) Angiotensin-(1-7)-stimulated nitric oxide and superoxide release from endothelial cells. Hypertension 37:72–76. [Abstract] [Google Scholar]
- Henrich WL, McAllister EA, Smith PB, Campbell WB (1988) Guanosine 3′,5′-cyclic monophosphate as a mediator of inhibition of renin release. Am J Physiol 255:F474–F478. [Abstract] [Google Scholar]
- Heringer-Walther S, Gembardt F, Perschel FH, Katz N, Schultheiss HP, Walther T (2012) The genetic deletion of Mas abolishes salt induced hypertension in mice. Eur J Pharmacol 689:147–153. [Abstract] [Google Scholar]
- Herrera M, Sparks MA, Alfonso-Pecchio AR, Harrison-Bernard LM, Coffman TM (2013) Lack of specificity of commercial antibodies leads to misidentification of angiotensin type 1 receptor protein. Hypertension 61:253–258. [Europe PMC free article] [Abstract] [Google Scholar]
- Heurich A, Hofmann-Winkler H, Gierer S, Liepold T, Jahn O, Pöhlmann S (2014) TMPRSS2 and ADAM17 cleave ACE2 differentially and only proteolysis by TMPRSS2 augments entry driven by the severe acute respiratory syndrome coronavirus spike protein. J Virol 88:1293–1307. [Europe PMC free article] [Abstract] [Google Scholar]
- Hilliard LM, Chow CL, Mirabito KM, Steckelings UM, Unger T, Widdop RE, Denton KM (2014) Angiotensin type 2 receptor stimulation increases renal function in female, but not male, spontaneously hypertensive rats. Hypertension 64:378–383. [Abstract] [Google Scholar]
- Hilliard LM, Jones ES, Steckelings UM, Unger T, Widdop RE, Denton KM (2012) Sex-specific influence of angiotensin type 2 receptor stimulation on renal function: a novel therapeutic target for hypertension. Hypertension 59:409–414. [Abstract] [Google Scholar]
- Hilliard LM, Nematbakhsh M, Kett MM, Teichman E, Sampson AK, Widdop RE, Evans RG, Denton KM (2011) Gender differences in pressure-natriuresis and renal autoregulation: role of the Angiotensin type 2 receptor. Hypertension 57:275–282. [Abstract] [Google Scholar]
- Hinrichs GR, Jensen BL, Svenningsen P (2020) Mechanisms of sodium retention in nephrotic syndrome. Curr Opin Nephrol Hypertens 29:207–212. [Abstract] [Google Scholar]
- Hollenberg NK, Fisher ND, Nussberger J, Moukarbel GV, Barkoudah E, Danser AH (2011) Renal responses to three types of renin-angiotensin system blockers in patients with diabetes mellitus on a high-salt diet: a need for higher doses in diabetic patients? J Hypertens 29:2454–2461. [Europe PMC free article] [Abstract] [Google Scholar]
- Hosojima MSato HYamamoto KKaseda RSoma TKobayashi ASuzuki AKabasawa HTakeyama AIkuyama K, et al. (2009) Regulation of megalin expression in cultured proximal tubule cells by angiotensin II type 1A receptor- and insulin-mediated signaling cross talk. Endocrinology 150:871–878. [Abstract] [Google Scholar]
- Huang J, Ledford KJ, Pitkin WB, Russo L, Najjar SM, Siragy HM (2013) Targeted deletion of murine CEACAM 1 activates PI3K-Akt signaling and contributes to the expression of (pro)renin receptor via CREB family and NF-κB transcription factors. Hypertension 62:317–323. [Europe PMC free article] [Abstract] [Google Scholar]
- Huang YYamamoto TMisaki TSuzuki HTogawa AOhashi NFukasawa HFujigaki YIchihara ANishiyama A, et al. (2012) Enhanced intrarenal receptor-mediated prorenin activation in chronic progressive anti-thymocyte serum nephritis rats on high salt intake. Am J Physiol Renal Physiol 303:F130–F138. [Abstract] [Google Scholar]
- Hudson M, Rahme E, Behlouli H, Sheppard R, Pilote L (2007) Sex differences in the effectiveness of angiotensin receptor blockers and angiotensin converting enzyme inhibitors in patients with congestive heart failure--a population study. Eur J Heart Fail 9:602–609. [Abstract] [Google Scholar]
- Ichihara AHayashi MKaneshiro YSuzuki FNakagawa TTada YKoura YNishiyama AOkada HUddin MN, et al. (2004) Inhibition of diabetic nephropathy by a decoy peptide corresponding to the “handle” region for nonproteolytic activation of prorenin. J Clin Invest 114:1128–1135. [Europe PMC free article] [Abstract] [Google Scholar]
- Ichihara A, Sakoda M, Kurauchi-Mito A, Nishiyama A, Itoh H (2008) Involvement of receptor-bound prorenin in development of nephropathy in diabetic db/db mice. J Am Soc Hypertens 2:332–340. [Abstract] [Google Scholar]
- Imai YKuba KRao SHuan YGuo FGuan BYang PSarao RWada TLeong-Poi H, et al. (2005) Angiotensin-converting enzyme 2 protects from severe acute lung failure. Nature 436:112–116. [Europe PMC free article] [Abstract] [Google Scholar]
- Ito S, Satoh M, Tamaki Y, Gotou H, Charney A, Okino N, Akahori M, Zhang J (2015) Safety and efficacy of LCZ696, a first-in-class angiotensin receptor neprilysin inhibitor, in Japanese patients with hypertension and renal dysfunction. Hypertens Res 38:269–275. [Europe PMC free article] [Abstract] [Google Scholar]
- Jafar THSchmid CHLanda MGiatras IToto RRemuzzi GMaschio GBrenner BMKamper AZucchelli P, et al. (2001) Angiotensin-converting enzyme inhibitors and progression of nondiabetic renal disease. A meta-analysis of patient-level data. Ann Intern Med 135:73–87. [Abstract] [Google Scholar]
- Jang HR, Jeon J, Park JH, Lee JE, Huh W, Oh HY, Kim YG (2014) Clinical relevance of urinary angiotensinogen and renin as potential biomarkers in patients with overt proteinuria. Transl Res 164:400–410. [Abstract] [Google Scholar]
- Jang HR, Kim SM, Lee YJ, Lee JE, Huh W, Kim DJ, Oh HY, Kim YG (2012) The origin and the clinical significance of urinary angiotensinogen in proteinuric IgA nephropathy patients. Ann Med 44:448–457. [Abstract] [Google Scholar]
- Jankowski VVanholder Rvan der Giet MTölle MKaradogan SGobom JFurkert JOksche AKrause ETran TN, et al. (2007) Mass-spectrometric identification of a novel angiotensin peptide in human plasma. Arterioscler Thromb Vasc Biol 27:297–302. [Abstract] [Google Scholar]
- Jeon J, Kim DH, Jang HR, Lee JE, Huh W, Kim HY, Kim DJ, Kim YG (2020) Urinary angiotensinogen as a surrogate marker predicting the antiproteinuric effects of angiotensin receptor blockers in patients with overt proteinuria: a multicenter prospective study. BMC Nephrol 21:180. [Europe PMC free article] [Abstract] [Google Scholar]
- Jeong JK, Diano S (2013) Prolyl carboxypeptidase and its inhibitors in metabolism. Trends Endocrinol Metab 24:61–67. [Europe PMC free article] [Abstract] [Google Scholar]
- Jing W, Vaziri ND, Nunes A, Suematsu Y, Farzaneh T, Khazaeli M, Moradi H (2017) LCZ696 (Sacubitril/valsartan) ameliorates oxidative stress, inflammation, fibrosis and improves renal function beyond angiotensin receptor blockade in CKD. Am J Transl Res 9:5473–5484. [Europe PMC free article] [Abstract] [Google Scholar]
- Joyner J, Neves L, Ferrario C, Brosnihan K (2008) Administration of D-alanine-[Ang-(1-7)] (A-779) prior to pregnancy in Sprague Dawley rats produces antidiuresis in late gestation. J Am Soc Hypertens 2:425–430. [Europe PMC free article] [Abstract] [Google Scholar]
- Kaltenecker CCDomenig OKopecky CAntlanger MPoglitsch MBerlakovich GKain RStegbauer JRahman MHellinger R, et al. (2020) Critical role of neprilysin in kidney angiotensin metabolism. Circ Res 127:593–606. [Abstract] [Google Scholar]
- Kamiyama M, Zsombok A, Kobori H (2012) Urinary angiotensinogen as a novel early biomarker of intrarenal renin-angiotensin system activation in experimental type 1 diabetes. J Pharmacol Sci 119:314–323. [Europe PMC free article] [Abstract] [Google Scholar]
- Kaneshiro Y, Ichihara A, Takemitsu T, Sakoda M, Suzuki F, Nakagawa T, Hayashi M, Inagami T (2006) Increased expression of cyclooxygenase-2 in the renal cortex of human prorenin receptor gene-transgenic rats. Kidney Int 70:641–646. [Abstract] [Google Scholar]
- Kang JJ, Toma I, Sipos A, Meer EJ, Vargas SL, Peti-Peterdi J (2008) The collecting duct is the major source of prorenin in diabetes. Hypertension 51:1597–1604. [Europe PMC free article] [Abstract] [Google Scholar]
- Kaplan BS, Restaino I, Raval DS, Gottlieb RP, Bernstein J (1994) Renal failure in the neonate associated with in utero exposure to non-steroidal anti-inflammatory agents. Pediatr Nephrol 8:700–704. [Abstract] [Google Scholar]
- Kastner C, Pohl M, Sendeski M, Stange G, Wagner CA, Jensen B, Patzak A, Bachmann S, Theilig F (2009) Effects of receptor-mediated endocytosis and tubular protein composition on volume retention in experimental glomerulonephritis. Am J Physiol Renal Physiol 296:F902–F911. [Abstract] [Google Scholar]
- Kemp BA, Bell JF, Rottkamp DM, Howell NL, Shao W, Navar LG, Padia SH, Carey RM (2012) Intrarenal angiotensin III is the predominant agonist for proximal tubule angiotensin type 2 receptors. Hypertension 60:387–395. [Europe PMC free article] [Abstract] [Google Scholar]
- Kemp BA, Howell NL, Gildea JJ, Keller SR, Padia SH, Carey RM (2014) AT2 receptor activation induces natriuresis and lowers blood pressure. Circ Res 115:388–399. [Europe PMC free article] [Abstract] [Google Scholar]
- Kemp BA, Howell NL, Keller SR, Gildea JJ, Padia SH, Carey RM (2016) AT2 receptor activation prevents sodium retention and reduces blood pressure in angiotensin II-dependent hypertension. Circ Res 119:532–543. [Europe PMC free article] [Abstract] [Google Scholar]
- Khan KN, Stanfield KM, Harris RK, Baron DA (2001) Expression of cyclooxygenase-2 in the macula densa of human kidney in hypertension, congestive heart failure, and diabetic nephropathy. Ren Fail 23:321–330. [Abstract] [Google Scholar]
- Kihara M, Umemura S, Sumida Y, Yokoyama N, Yabana M, Nyui N, Tamura K, Murakami K, Fukamizu A, Ishii M (1998) Genetic deficiency of angiotensinogen produces an impaired urine concentrating ability in mice. Kidney Int 53:548–555. [Abstract] [Google Scholar]
- Kim HPark SJhee JHYun HRPark JTHan SHLee JKim SWKim YHOh YK, et al. ; KNOW-CKD Investigators Group (2019) Urinary angiotensinogen level is associated with potassium homeostasis and clinical outcome in patients with polycystic kidney disease: a prospective cohort study. BMC Nephrol 20:104. [Europe PMC free article] [Abstract] [Google Scholar]
- Kim SM, Chen L, Mizel D, Huang YG, Briggs JP, Schnermann J (2007) Low plasma renin and reduced renin secretory responses to acute stimuli in conscious COX-2-deficient mice. Am J Physiol Renal Physiol 292:F415–F422. [Abstract] [Google Scholar]
- Kim SM, Jang HR, Lee YJ, Lee JE, Huh WS, Kim DJ, Oh HY, Kim YG (2011a) Urinary angiotensinogen levels reflect the severity of renal histopathology in patients with chronic kidney disease. Clin Nephrol 76:117–123. [Abstract] [Google Scholar]
- Kim YG, Song SB, Lee SH, Moon JY, Jeong KH, Lee TW, Ihm CG (2011b) Urinary angiotensinogen as a predictive marker in patients with immunoglobulin A nephropathy. Clin Exp Nephrol 15:720–726. [Abstract] [Google Scholar]
- Kinouchi KIchihara ASano MSun-Wada GHWada YKurauchi-Mito ABokuda KNarita TOshima YSakoda M, et al. (2010) The (pro)renin receptor/ATP6AP2 is essential for vacuolar H+-ATPase assembly in murine cardiomyocytes. Circ Res 107:30–34. [Abstract] [Google Scholar]
- Klotz S, Burkhoff D, Garrelds IM, Boomsma F, Danser AH (2009) The impact of left ventricular assist device-induced left ventricular unloading on the myocardial renin-angiotensin-aldosterone system: therapeutic consequences? Eur Heart J 30:805–812. [Abstract] [Google Scholar]
- Kobori H, Katsurada A, Ozawa Y, Satou R, Miyata K, Hase N, Suzaki Y, Shoji T (2007a) Enhanced intrarenal oxidative stress and angiotensinogen in IgA nephropathy patients. Biochem Biophys Res Commun 358:156–163. [Europe PMC free article] [Abstract] [Google Scholar]
- Kobori H, Nangaku M, Navar LG, Nishiyama A (2007b) The intrarenal renin-angiotensin system: from physiology to the pathobiology of hypertension and kidney disease. Pharmacol Rev 59:251–287. [Abstract] [Google Scholar]
- Kobori H, Ohashi N, Katsurada A, Miyata K, Satou R, Saito T, Yamamoto T (2008) Urinary angiotensinogen as a potential biomarker of severity of chronic kidney diseases. J Am Soc Hypertens 2:349–354. [Europe PMC free article] [Abstract] [Google Scholar]
- Kocyigit I, Yilmaz MI, Unal A, Ozturk F, Eroglu E, Yazici C, Orscelik O, Sipahioglu MH, Tokgoz B, Oymak O (2013) A link between the intrarenal renin angiotensin system and hypertension in autosomal dominant polycystic kidney disease. Am J Nephrol 38:218–225. [Abstract] [Google Scholar]
- Koizumi M, Ueda K, Niimura F, Nishiyama A, Yanagita M, Saito A, Pastan I, Fujita T, Fukagawa M, Matsusaka T (2019) Podocyte injury augments intrarenal angiotensin II generation and sodium retention in a megalin-dependent manner. Hypertension 74:509–517. [Europe PMC free article] [Abstract] [Google Scholar]
- Koka V, Huang XR, Chung AC, Wang W, Truong LD, Lan HY (2008) Angiotensin II up-regulates angiotensin I-converting enzyme (ACE), but down-regulates ACE2 via the AT1-ERK/p38 MAP kinase pathway. Am J Pathol 172:1174–1183. [Europe PMC free article] [Abstract] [Google Scholar]
- Kömhoff M, Jeck ND, Seyberth HW, Gröne HJ, Nüsing RM, Breyer MD (2000) Cyclooxygenase-2 expression is associated with the renal macula densa of patients with Bartter-like syndrome. Kidney Int 58:2420–2424. [Abstract] [Google Scholar]
- Kong YZhao XQiu MLin YFeng PLi SLiang BZhu QHuang HLi C, et al. (2021) Tubular Mas receptor mediates lipid-induced kidney injury. Cell Death Dis 12:110. [Europe PMC free article] [Abstract] [Google Scholar]
- Konishi YNishiyama AMorikawa TKitabayashi CShibata MHamada MKishida MHitomi HKiyomoto HMiyashita T, et al. (2011) Relationship between urinary angiotensinogen and salt sensitivity of blood pressure in patients with IgA nephropathy. Hypertension 58:205–211. [Europe PMC free article] [Abstract] [Google Scholar]
- Konoshita TWakahara SMizuno SMotomura MAoyama CMakino YKawai YKato NKoni IMiyamori I, et al. (2006) Tissue gene expression of renin-angiotensin system in human type 2 diabetic nephropathy. Diabetes Care 29:848–852. [Abstract] [Google Scholar]
- Kostenis EMilligan GChristopoulos ASanchez-Ferrer CFHeringer-Walther SSexton PMGembardt FKellett EMartini LVanderheyden P, et al. (2005) G-protein-coupled receptor Mas is a physiological antagonist of the angiotensin II type 1 receptor. Circulation 111:1806–1813. [Abstract] [Google Scholar]
- Krause LMH, Kemp BA, Tan ASJ, Jones ES, Del Borgo MP, Aguilar MI, Denton KM, Carey RM, Widdop RE (2020) Renal functional effects of the highly selective AT2R agonist, β-Pro7 Ang III, in normotensive rats. Clin Sci (Lond) 134:871–884. [Europe PMC free article] [Abstract] [Google Scholar]
- Krop M, Danser AH (2008) Circulating versus tissue renin-angiotensin system: on the origin of (pro)renin. Curr Hypertens Rep 10:112–118. [Abstract] [Google Scholar]
- Kuba KImai YRao SGao HGuo FGuan BHuan YYang PZhang YDeng W, et al. (2005) A crucial role of angiotensin converting enzyme 2 (ACE2) in SARS coronavirus-induced lung injury. Nat Med 11:875–879. [Europe PMC free article] [Abstract] [Google Scholar]
- Kukida M, Cai L, Ye D, Sawada H, Katsumata Y, Franklin MK, Hecker PI, Campbell KS, Danser AHJ, Mullick AE, Daugherty A, Temel RE, Lu HS (2021) Renal angiotensinogen is predominantly liver derived in nonhuman primates. Arterioscler Thromb Vasc Biol 41:2851–2853 DOI: 10.1161/ATVBAHA.121.316590. [Europe PMC free article] [Abstract] [Google Scholar]
- Kurultak I, Sengul S, Kocak S, Erdogmus S, Calayoglu R, Mescigil P, Keven K, Erturk S, Erbay B, Duman N (2014) Urinary angiotensinogen, related factors and clinical implications in normotensive autosomal dominant polycystic kidney disease patients. Ren Fail 36:717–721. [Abstract] [Google Scholar]
- Kutlugün AA, Altun B, Aktan U, Turkmen E, Altindal M, Yildirim T, Yilmaz R, Arici M, Erdem Y, Turgan C (2012) The relation between urinary angiotensinogen and proteinuria in renal AA amyloidosis patients. Amyloid 19:28–32. [Abstract] [Google Scholar]
- Lai KN, Leung JC, Lai KB, To WY, Yeung VT, Lai FM (1998) Gene expression of the renin-angiotensin system in human kidney. J Hypertens 16:91–102. [Abstract] [Google Scholar]
- Lambert DW, Yarski M, Warner FJ, Thornhill P, Parkin ET, Smith AI, Hooper NM, Turner AJ (2005) Tumor necrosis factor-α convertase (ADAM17) mediates regulated ectodomain shedding of the severe-acute respiratory syndrome-coronavirus (SARS-CoV) receptor, angiotensin-converting enzyme-2 (ACE2). J Biol Chem 280:30113–30119. [Europe PMC free article] [Abstract] [Google Scholar]
- Lansang MC, Price DA, Laffel LM, Osei SY, Fisher ND, Erani D, Hollenberg NK (2001) Renal vascular responses to captopril and to candesartan in patients with type 1 diabetes mellitus. Kidney Int 59:1432–1438. [Abstract] [Google Scholar]
- Lautner RQVillela DCFraga-Silva RASilva NVerano-Braga TCosta-Fraga FJankowski JJankowski VSousa FAlzamora A, et al. (2013) Discovery and characterization of alamandine: a novel component of the renin-angiotensin system. Circ Res 112:1104–1111. [Abstract] [Google Scholar]
- Lawrence AC, Evin G, Kladis A, Campbell DJ (1990) An alternative strategy for the radioimmunoassay of angiotensin peptides using amino-terminal-directed antisera: measurement of eight angiotensin peptides in human plasma. J Hypertens 8:715–724. [Abstract] [Google Scholar]
- Lee MJKim SSKim IJSong SHKim EHSeo JYKim JHKim SJeon YKKim BH, et al. (2017) Changes in urinary angiotensinogen associated with deterioration of kidney function in patients with type 2 diabetes mellitus. J Korean Med Sci 32:782–788. [Europe PMC free article] [Abstract] [Google Scholar]
- Lee YJ, Cho S, Kim SR, Jang HR, Lee JE, Huh W, Kim DJ, Oh HY, Kim YG (2011) Effect of losartan on proteinuria and urinary angiotensinogen excretion in non-diabetic patients with chronic kidney disease. Postgrad Med J 87:664–669. [Abstract] [Google Scholar]
- Leehey DJ, Singh AK, Bast JP, Sethupathi P, Singh R (2008) Glomerular renin angiotensin system in streptozotocin diabetic and Zucker diabetic fatty rats. Transl Res 151:208–216. [Abstract] [Google Scholar]
- Lely A, Hamming I, van Goor H, Navis G (2004) Renal ACE2 expression in human kidney disease. J Pathol 204:587–593. [Abstract] [Google Scholar]
- Lewis EJ, Hunsicker LG, Bain RP, Rohde RD; The Collaborative Study Group (1993) The effect of angiotensin-converting-enzyme inhibition on diabetic nephropathy. N Engl J Med 329:1456–1462. [Abstract] [Google Scholar]
- Li C, Culver SA, Quadri S, Ledford KL, Al-Share QY, Ghadieh HE, Najjar SM, Siragy HM (2015) High-fat diet amplifies renal renin angiotensin system expression, blood pressure elevation, and renal dysfunction caused by Ceacam1 null deletion. Am J Physiol Endocrinol Metab 309:E802–E810. [Europe PMC free article] [Abstract] [Google Scholar]
- Li LKonishi YMorikawa TZhang YKitabayashi CKobara HMasaki TNakano DHitomi HKobori H, et al. (2018a) Effect of a SGLT2 inhibitor on the systemic and intrarenal renin-angiotensin system in subtotally nephrectomized rats. J Pharmacol Sci 137:220–223. [Europe PMC free article] [Abstract] [Google Scholar]
- Li P, Chappell MC, Ferrario CM, Brosnihan KB (1997) Angiotensin-(1-7) augments bradykinin-induced vasodilation by competing with ACE and releasing nitric oxide. Hypertension 29:394–400. [Abstract] [Google Scholar]
- Li XC, Cook JL, Rubera I, Tauc M, Zhang F, Zhuo JL (2011) Intrarenal transfer of an intracellular fluorescent fusion of angiotensin II selectively in proximal tubules increases blood pressure in rats and mice. Am J Physiol Renal Physiol 300:F1076–F1088. [Europe PMC free article] [Abstract] [Google Scholar]
- Li XC, Hopfer U, Zhuo JL (2009) AT1 receptor-mediated uptake of angiotensin II and NHE-3 expression in proximal tubule cells through a microtubule-dependent endocytic pathway. Am J Physiol Renal Physiol 297:F1342–F1352. [Europe PMC free article] [Abstract] [Google Scholar]
- Li XC, Leite APO, Zheng X, Zhao C, Chen X, Zhang L, Zhou X, Rubera I, Tauc M, Zhuo JL (2021) Proximal tubule-specific deletion of angiotensin II type 1a receptors in the kidney attenuates circulating and intratubular angiotensin II-induced hypertension in PT-Agtr1a-/- mice. Hypertension 77:1285–1298. [Europe PMC free article] [Abstract] [Google Scholar]
- Li XC, Navar LG, Shao Y, Zhuo JL (2007) Genetic deletion of AT1a receptors attenuates intracellular accumulation of ANG II in the kidney of AT1a receptor-deficient mice. Am J Physiol Renal Physiol 293:F586–F593. [Europe PMC free article] [Abstract] [Google Scholar]
- Li XC, Zhou X, Zhuo JL (2020) Evidence for a physiological mitochondrial angiotensin II system in the kidney proximal tubules: novel roles of mitochondrial Ang II/AT1a/O2- and Ang II/AT2/NO signaling. Hypertension 76:121–132. [Europe PMC free article] [Abstract] [Google Scholar]
- Li XC, Zhu D, Zheng X, Zhang J, Zhuo JL (2018b) Intratubular and intracellular renin-angiotensin system in the kidney: a unifying perspective in blood pressure control. Clin Sci (Lond) 132:1383–1401. [Europe PMC free article] [Abstract] [Google Scholar]
- Li XC, Zhuo JL (2008) Intracellular ANG II directly induces in vitro transcription of TGF-beta1, MCP-1, and NHE-3 mRNAs in isolated rat renal cortical nuclei via activation of nuclear AT1a receptors. Am J Physiol Cell Physiol 294:C1034–C1045. [Europe PMC free article] [Abstract] [Google Scholar]
- Li XC, Zhuo JL (2011) Phosphoproteomic analysis of AT1 receptor-mediated signaling responses in proximal tubules of angiotensin II-induced hypertensive rats. Kidney Int 80:620–632. [Europe PMC free article] [Abstract] [Google Scholar]
- Li XC, Zhuo JL (2013) Proximal tubule-dominant transfer of AT(1a) receptors induces blood pressure responses to intracellular angiotensin II in AT(1a) receptor-deficient mice. Am J Physiol Regul Integr Comp Physiol 304:R588–R598. [Europe PMC free article] [Abstract] [Google Scholar]
- Lin EE, Sequeira-Lopez ML, Gomez RA (2014) RBP-J in FOXD1+ renal stromal progenitors is crucial for the proper development and assembly of the kidney vasculature and glomerular mesangial cells. Am J Physiol Renal Physiol 306:F249–F258. [Europe PMC free article] [Abstract] [Google Scholar]
- Liu CXHu QWang YZhang WMa ZYFeng JBWang RWang XPDong BGao F, et al. (2011a) Angiotensin-converting enzyme (ACE) 2 overexpression ameliorates glomerular injury in a rat model of diabetic nephropathy: a comparison with ACE inhibition. Mol Med 17:59–69. [Europe PMC free article] [Abstract] [Google Scholar]
- Liu L, Gonzalez AA, McCormack M, Seth DM, Kobori H, Navar LG, Prieto MC (2011b) Increased renin excretion is associated with augmented urinary angiotensin II levels in chronic angiotensin II-infused hypertensive rats. Am J Physiol Renal Physiol 301:F1195–F1201. [Europe PMC free article] [Abstract] [Google Scholar]
- Liu Z, Huang XR, Chen HY, Penninger JM, Lan HY (2012) Loss of angiotensin-converting enzyme 2 enhances TGF-β/Smad-mediated renal fibrosis and NF-κB-driven renal inflammation in a mouse model of obstructive nephropathy. Lab Invest 92:650–661. [Abstract] [Google Scholar]
- Lores E, Wysocki J, Batlle D (2020) ACE2, the kidney and the emergence of COVID-19 two decades after ACE2 discovery. Clin Sci (Lond) 134:2791–2805. [Abstract] [Google Scholar]
- Lu X, Garrelds IM, Wagner CA, Danser AH, Meima ME (2013) (Pro)renin receptor is required for prorenin-dependent and -independent regulation of vacuolar H+-ATPase activity in MDCK.C11 collecting duct cells. Am J Physiol Renal Physiol 305:F417–F425. [Abstract] [Google Scholar]
- Lu X, Wang F, Liu M, Yang KT, Nau A, Kohan DE, Reese V, Richardson RS, Yang T (2016a) Activation of ENaC in collecting duct cells by prorenin and its receptor PRR: involvement of Nox4-derived hydrogen peroxide. Am J Physiol Renal Physiol 310:F1243–F1250. [Europe PMC free article] [Abstract] [Google Scholar]
- Lu XWang FXu CSoodvilai SPeng KSu JZhao LYang KTFeng YZhou SF, et al. (2016b) Soluble (pro)renin receptor via β-catenin enhances urine concentration capability as a target of liver X receptor. Proc Natl Acad Sci USA 113:E1898–E1906. [Europe PMC free article] [Abstract] [Google Scholar]
- Ludwig J, Kerscher S, Brandt U, Pfeiffer K, Getlawi F, Apps DK, Schägger H (1998) Identification and characterization of a novel 9.2-kDa membrane sector-associated protein of vacuolar proton-ATPase from chromaffin granules. J Biol Chem 273:10939–10947. [Abstract] [Google Scholar]
- Luetscher JA, Kraemer FB, Wilson DM, Schwartz HC, Bryer-Ash M (1985) Increased plasma inactive renin in diabetes mellitus. A marker of microvascular complications. N Engl J Med 312:1412–1417. [Abstract] [Google Scholar]
- Maier CSchadock IHaber PKWysocki JYe MKanwar YFlask CAYu XHoit BDAdams GN, et al. (2017) Prolylcarboxypeptidase deficiency is associated with increased blood pressure, glomerular lesions, and cardiac dysfunction independent of altered circulating and cardiac angiotensin II. J Mol Med (Berl) 95:473–486. [Abstract] [Google Scholar]
- Marahrens B, Schulze A, Wysocki J, Lin M-H, Ye M, Kanwar YS, Bader M, Velez JCQ, Miner JH, Batlle D (2021) Knockout of aminopeptidase A in mice causes functional alterations and morphological glomerular basement membrane changes in the kidneys. Kidney Int 99:900–913. [Abstract] [Google Scholar]
- Markovič T, Jakopin Ž, Dolenc MS, Mlinarič-Raščan I (2017) Structural features of subtype-selective EP receptor modulators. Drug Discov Today 22:57–71. [Abstract] [Google Scholar]
- Marquez A, Wysocki J, Pandit J, Batlle D (2021) An update on ACE2 amplification and its therapeutic potential. Acta Physiol (Oxf) 231:e13513. [Europe PMC free article] [Abstract] [Google Scholar]
- Martínez JM, Prieto I, Ramírez MJ, de Gasparo M, Hermoso F, Arias JM, Alba F, Ramírez M (1998) Sex differences and age-related changes in human serum aminopeptidase A activity. Clin Chim Acta 274:53–61. [Abstract] [Google Scholar]
- Matavelli LC, Huang J, Siragy HM (2010) (Pro)renin receptor contributes to diabetic nephropathy by enhancing renal inflammation. Clin Exp Pharmacol Physiol 37:277–282. [Europe PMC free article] [Abstract] [Google Scholar]
- Matavelli LC, Huang J, Siragy HM (2011) Angiotensin AT2 receptor stimulation inhibits early renal inflammation in renovascular hypertension. Hypertension 57:308–313. [Europe PMC free article] [Abstract] [Google Scholar]
- Matsubara HSugaya TMurasawa SNozawa YMori YMasaki HMaruyama KTsutumi YShibasaki YMoriguchi Y, et al. (1998) Tissue-specific expression of human angiotensin II AT1 and AT2 receptors and cellular localization of subtype mRNAs in adult human renal cortex using in situ hybridization. Nephron 80:25–34. [Abstract] [Google Scholar]
- Matsusaka T, Niimura F, Pastan I, Shintani A, Nishiyama A, Ichikawa I (2014) Podocyte injury enhances filtration of liver-derived angiotensinogen and renal angiotensin II generation. Kidney Int 85:1068–1077. [Europe PMC free article] [Abstract] [Google Scholar]
- Matsusaka T, Niimura F, Shimizu A, Pastan I, Saito A, Kobori H, Nishiyama A, Ichikawa I (2012) Liver angiotensinogen is the primary source of renal angiotensin II. J Am Soc Nephrol 23:1181–1189. [Europe PMC free article] [Abstract] [Google Scholar]
- Matsuyama T, Ohashi N, Aoki T, Ishigaki S, Isobe S, Sato T, Fujikura T, Kato A, Miyajima H, Yasuda H (2021) Circadian rhythm of the intrarenal renin-angiotensin system is caused by glomerular filtration of liver-derived angiotensinogen depending on glomerular capillary pressure in adriamycin nephropathy rats. Hypertens Res 44:618–627. [Abstract] [Google Scholar]
- Mc Causland FRLefkowitz MPClaggett BAnavekar NSSenni MGori MJhund PSMcGrath MMPacker MShi V, et al. (2020) Angiotensin-neprilysin inhibition and renal outcomes in heart failure with preserved ejection fraction. Circulation 142:1236–1245. [Abstract] [Google Scholar]
- McDonald JK, Zeitman BB, Callahan PX, Ellis S (1974) Angiotensinase activity of dipeptidyl aminopeptidase I (cathepsin C) of rat liver. J Biol Chem 249:234–240. [Abstract] [Google Scholar]
- McMurray JJVPacker MDesai ASGong JLefkowitz MPRizkala ARRouleau JLShi VCSolomon SDSwedberg K, et al. ; PARADIGM-HF Investigators and Committees (2014) Angiotensin-neprilysin inhibition versus enalapril in heart failure. N Engl J Med 371:993–1004. [Abstract] [Google Scholar]
- Meima ME, Danser AH (2011) The prorenin receptor: what’s in a name. J Am Soc Nephrol 22:2141–2143. [Abstract] [Google Scholar]
- Metzger R, Bohle RM, Pauls K, Eichner G, Alhenc-Gelas F, Danilov SM, Franke FE (1999) Angiotensin-converting enzyme in non-neoplastic kidney diseases. Kidney Int 56:1442–1454. [Abstract] [Google Scholar]
- Metzger R, Franke FE, Bohle RM, Alhenc-Gelas F, Danilov SM (2011) Heterogeneous distribution of angiotensin I-converting enzyme (CD143) in the human and rat vascular systems: vessel, organ and species specificity. Microvasc Res 81:206–215. [Abstract] [Google Scholar]
- Mezzano S, Droguett A, Burgos ME, Ardiles LG, Flores CA, Aros CA, Caorsi I, Vío CP, Ruiz-Ortega M, Egido J (2003) Renin-angiotensin system activation and interstitial inflammation in human diabetic nephropathy. Kidney Int Suppl 64 (Suppl 86):S64–S70. [Abstract] [Google Scholar]
- Miller JA, Cherney DZ, Duncan JA, Lai V, Burns KD, Kennedy CR, Zimpelmann J, Gao W, Cattran DC, Scholey JW (2006) Gender differences in the renal response to renin-angiotensin system blockade. J Am Soc Nephrol 17:2554–2560. [Abstract] [Google Scholar]
- Mills KTKobori HHamm LLAlper ABKhan IERahman MNavar LGLiu YBrowne GMBatuman V, et al. (2012) Increased urinary excretion of angiotensinogen is associated with risk of chronic kidney disease. Nephrol Dial Transplant 27:3176–3181. [Europe PMC free article] [Abstract] [Google Scholar]
- Mirabito KM, Hilliard LM, Kett MM, Brown RD, Booth SC, Widdop RE, Moritz KM, Evans RG, Denton KM (2014) Sex- and age-related differences in the chronic pressure-natriuresis relationship: role of the angiotensin type 2 receptor. Am J Physiol Renal Physiol 307:F901–F907. [Abstract] [Google Scholar]
- Mitsui TNomura SOkada MOhno YKobayashi HNakashima YMurata YTakeuchi MKuno NNagasaka T, et al. (2003) Hypertension and angiotensin II hypersensitivity in aminopeptidase A-deficient mice. Mol Med 9:57–62. [Europe PMC free article] [Abstract] [Google Scholar]
- Miyata K, Ohashi N, Suzaki Y, Katsurada A, Kobori H (2008) Sequential activation of the reactive oxygen species/angiotensinogen/renin-angiotensin system axis in renal injury of type 2 diabetic rats. Clin Exp Pharmacol Physiol 35:922–927. [Europe PMC free article] [Abstract] [Google Scholar]
- Miyata KNLo CSZhao SLiao MCPang YChang SYPeng JKretzler MFilep JGIngelfinger JR, et al. (2021) Angiotensin II up-regulates sodium-glucose co-transporter 2 expression and SGLT2 inhibitor attenuates Ang II-induced hypertensive renal injury in mice. Clin Sci (Lond) 135:943–961. [Europe PMC free article] [Abstract] [Google Scholar]
- Miyata N, Park F, Li XF, Cowley AW Jr (1999) Distribution of angiotensin AT1 and AT2 receptor subtypes in the rat kidney. Am J Physiol 277:F437–F446. [Abstract] [Google Scholar]
- Mizuiri SHemmi HArita MOhashi YTanaka YMiyagi MSakai KIshikawa YShibuya KHase H, et al. (2008) Expression of ACE and ACE2 in individuals with diabetic kidney disease and healthy controls. Am J Kidney Dis 51:613–623. [Abstract] [Google Scholar]
- Mohamed TH, Watanabe H, Kaur R, Belyea BC, Walker PD, Gomez RA, Sequeira-Lopez MLS (2020) Renin-expressing cells require β1-integrin for survival and for development and maintenance of the renal vasculature. Hypertension 76:458–467. [Europe PMC free article] [Abstract] [Google Scholar]
- Monteil V, Kwon H, Prado P, Hagelkrüys A, Wimmer RA, Stahl M, Leopoldi A, Garreta E, Del Pozo CH, Prosper F (2020) Inhibition of SARS-CoV-2 infections in engineered human tissues using clinical-grade soluble human ACE2. Cell 181:905–913.e7. [Europe PMC free article] [Abstract] [Google Scholar]
- Morham SGLangenbach RLoftin CDTiano HFVouloumanos NJennette JCMahler JFKluckman KDLedford ALee CA, et al. (1995) Prostaglandin synthase 2 gene disruption causes severe renal pathology in the mouse. Cell 83:473–482. [Abstract] [Google Scholar]
- Morimoto S, Ando T, Niiyama M, Seki Y, Yoshida N, Watanabe D, Kawakami-Mori F, Kobori H, Nishiyama A, Ichihara A (2014) Serum soluble (pro)renin receptor levels in patients with essential hypertension. Hypertens Res 37:642–648. [Europe PMC free article] [Abstract] [Google Scholar]
- Morizane R, Lam AQ, Freedman BS, Kishi S, Valerius MT, Bonventre JV (2015) Nephron organoids derived from human pluripotent stem cells model kidney development and injury. Nat Biotechnol 33:1193–1200. [Europe PMC free article] [Abstract] [Google Scholar]
- Murphy TJ, Alexander RW, Griendling KK, Runge MS, Bernstein KE (1991) Isolation of a cDNA encoding the vascular type-1 angiotensin II receptor. Nature 351:233–236. [Abstract] [Google Scholar]
- Nadarajah R, Milagres R, Dilauro M, Gutsol A, Xiao F, Zimpelmann J, Kennedy C, Wysocki J, Batlle D, Burns KD (2012) Podocyte-specific overexpression of human angiotensin-converting enzyme 2 attenuates diabetic nephropathy in mice. Kidney Int 82:292–303. [Europe PMC free article] [Abstract] [Google Scholar]
- Nagai YYao LKobori HMiyata KOzawa YMiyatake AYukimura TShokoji TKimura SKiyomoto H, et al. (2005) Temporary angiotensin II blockade at the prediabetic stage attenuates the development of renal injury in type 2 diabetic rats. J Am Soc Nephrol 16:703–711. [Europe PMC free article] [Abstract] [Google Scholar]
- Nakagawa T, Suzuki-Nakagawa C, Watanabe A, Asami E, Matsumoto M, Nakano M, Ebihara A, Uddin MN, Suzuki F (2017) Site-1 protease is required for the generation of soluble (pro)renin receptor. J Biochem 161:369–379. [Abstract] [Google Scholar]
- Nakano D, Kobori H, Burford JL, Gevorgyan H, Seidel S, Hitomi H, Nishiyama A, Peti-Peterdi J (2012) Multiphoton imaging of the glomerular permeability of angiotensinogen. J Am Soc Nephrol 23:1847–1856. [Europe PMC free article] [Abstract] [Google Scholar]
- Nasrallah R, Hassouneh R, Hébert RL (2014) Chronic kidney disease: targeting prostaglandin E2 receptors. Am J Physiol Renal Physiol 307:F243–F250. [Abstract] [Google Scholar]
- Navar LG, Harrison-Bernard LM, Nishiyama A, Kobori H (2002) Regulation of intrarenal angiotensin II in hypertension. Hypertension 39:316–322. [Europe PMC free article] [Abstract] [Google Scholar]
- Nguyen G, Delarue F, Burcklé C, Bouzhir L, Giller T, Sraer JD (2002) Pivotal role of the renin/prorenin receptor in angiotensin II production and cellular responses to renin. J Clin Invest 109:1417–1427. [Europe PMC free article] [Abstract] [Google Scholar]
- Nguyen G, Muller DN (2010) The biology of the (pro)renin receptor. J Am Soc Nephrol 21:18–23. [Abstract] [Google Scholar]
- Ni J, Yang F, Huang XR, Meng J, Chen J, Bader M, Penninger JM, Fung E, Yu XQ, Lan HY (2020) Dual deficiency of angiotensin-converting enzyme-2 and Mas receptor enhances angiotensin II-induced hypertension and hypertensive nephropathy. J Cell Mol Med 24:13093–13103. [Europe PMC free article] [Abstract] [Google Scholar]
- Niimura FLabosky PAKakuchi JOkubo SYoshida HOikawa TIchiki TNaftilan AJFogo AInagami T, et al. (1995) Gene targeting in mice reveals a requirement for angiotensin in the development and maintenance of kidney morphology and growth factor regulation. J Clin Invest 96:2947–2954. [Europe PMC free article] [Abstract] [Google Scholar]
- Nishiyama A, Kobori H (2018) Independent regulation of renin-angiotensin-aldosterone system in the kidney. Clin Exp Nephrol 22:1231–1239. [Europe PMC free article] [Abstract] [Google Scholar]
- Nishiyama AKonishi YOhashi NMorikawa TUrushihara MMaeda IHamada MKishida MHitomi HShirahashi N, et al. (2011) Urinary angiotensinogen reflects the activity of intrarenal renin-angiotensin system in patients with IgA nephropathy. Nephrol Dial Transplant 26:170–177. [Europe PMC free article] [Abstract] [Google Scholar]
- Nishiyama ANakagawa TKobori HNagai YOkada NKonishi YMorikawa TOkumura MMeda IKiyomoto H, et al. (2008) Strict angiotensin blockade prevents the augmentation of intrarenal angiotensin II and podocyte abnormalities in type 2 diabetic rats with microalbuminuria. J Hypertens 26:1849–1859. [Europe PMC free article] [Abstract] [Google Scholar]
- Odya CE, Marinkovic DV, Hammon KJ, Stewart TA, Erdös EG (1978) Purification and properties of prolylcarboxypeptidase (angiotensinase C) from human kidney. J Biol Chem 253:5927–5931. [Abstract] [Google Scholar]
- Ohashi N, Aoki T, Matsuyama T, Ishigaki S, Isobe S, Katahashi N, Sato T, Fujikura T, Kato A, Yasuda H (2020) The urinary angiotensinogen to urinary albumin ratio reflects whether the renin-angiotensin system in the kidney Is activated due to filtration of plasma angiotensinogen through the damaged glomeruli or the production of angiotensinogen in the proximal tubules. Intern Med 59:357–364. [Europe PMC free article] [Abstract] [Google Scholar]
- Ohashi N, Isobe S, Ishigaki S, Suzuki T, Ono M, Fujikura T, Tsuji T, Kato A, Ozono S, Yasuda H (2017) Intrarenal renin-angiotensin system activity is augmented after initiation of dialysis. Hypertens Res 40:364–370. [Abstract] [Google Scholar]
- Ohashi NYamamoto THuang YMisaki TFukasawa HSuzuki HTogawa ASuzuki SFujigaki YNakagawa T, et al. (2008) Intrarenal RAS activity and urinary angiotensinogen excretion in anti-thymocyte serum nephritis rats. Am J Physiol Renal Physiol 295:F1512–F1518. [Abstract] [Google Scholar]
- Oishi Y, Ozono R, Yano Y, Teranishi Y, Akishita M, Horiuchi M, Oshima T, Kambe M (2003) Cardioprotective role of AT2 receptor in postinfarction left ventricular remodeling. Hypertension 41:814–818. [Abstract] [Google Scholar]
- Oka M, Medrano S, Sequeira-Lόpez MLS, Gómez RA (2017) Chronic stimulation of renin cells leads to vascular pathology. Hypertension 70:119–128. [Europe PMC free article] [Abstract] [Google Scholar]
- Okabe M, Miyazaki Y, Niimura F, Pastan I, Nishiyama A, Yokoo T, Ichikawa I, Matsusaka T (2015) Unilateral ureteral obstruction attenuates intrarenal angiotensin II generation induced by podocyte injury. Am J Physiol Renal Physiol 308:F932–F937. [Europe PMC free article] [Abstract] [Google Scholar]
- Oshima YKinouchi KIchihara ASakoda MKurauchi-Mito ABokuda KNarita TKurosawa HSun-Wada GHWada Y, et al. (2011) Prorenin receptor is essential for normal podocyte structure and function. J Am Soc Nephrol 22:2203–2212. [Europe PMC free article] [Abstract] [Google Scholar]
- Packer M, Claggett B, Lefkowitz MP, McMurray JJV, Rouleau JL, Solomon SD, Zile MR (2018) Effect of neprilysin inhibition on renal function in patients with type 2 diabetes and chronic heart failure who are receiving target doses of inhibitors of the renin-angiotensin system: a secondary analysis of the PARADIGM-HF trial. Lancet Diabetes Endocrinol 6:547–554. [Abstract] [Google Scholar]
- Padia SH, Carey RM (2013) AT2 receptors: beneficial counter-regulatory role in cardiovascular and renal function. Pflugers Arch 465:99–110. [Europe PMC free article] [Abstract] [Google Scholar]
- Padia SH, Kemp BA, Howell NL, Fournie-Zaluski MC, Roques BP, Carey RM (2008) Conversion of renal angiotensin II to angiotensin III is critical for AT2 receptor-mediated natriuresis in rats. Hypertension 51:460–465. [Abstract] [Google Scholar]
- Padia SH, Kemp BA, Howell NL, Gildea JJ, Keller SR, Carey RM (2009) Intrarenal angiotensin III infusion induces natriuresis and angiotensin type 2 receptor translocation in Wistar-Kyoto but not in spontaneously hypertensive rats. Hypertension 53:338–343. [Europe PMC free article] [Abstract] [Google Scholar]
- Padia SH, Kemp BA, Howell NL, Siragy HM, Fournie-Zaluski MC, Roques BP, Carey RM (2007) Intrarenal aminopeptidase N inhibition augments natriuretic responses to angiotensin III in angiotensin type 1 receptor-blocked rats. Hypertension 49:625–630. [Abstract] [Google Scholar]
- Palmer SC, Mavridis D, Navarese E, Craig JC, Tonelli M, Salanti G, Wiebe N, Ruospo M, Wheeler DC, Strippoli GF (2015) Comparative efficacy and safety of blood pressure-lowering agents in adults with diabetes and kidney disease: a network meta-analysis. Lancet 385:2047–2056. [Abstract] [Google Scholar]
- Pang PSButler JCollins SPCotter GDavison BAEzekowitz JAFilippatos GLevy PDMetra MPonikowski P, et al. (2017) Biased ligand of the angiotensin II type 1 receptor in patients with acute heart failure: a randomized, double-blind, placebo-controlled, phase IIB, dose ranging trial (BLAST-AHF). Eur Heart J 38:2364–2373. [Europe PMC free article] [Abstract] [Google Scholar]
- Pang X, Shimizu A, Kurita S, Zankov DP, Takeuchi K, Yasuda-Yamahara M, Kume S, Ishida T, Ogita H (2016) Novel therapeutic role for dipeptidyl peptidase III in the treatment of hypertension. Hypertension 68:630–641. [Abstract] [Google Scholar]
- Park HCKang AYJang JYKim HHan MOh KHKim SHNoh JWCheong HIHwang YH, et al. (2015a) Increased urinary angiotensinogen/creatinine (AGT/Cr) ratio may be associated with reduced renal function in autosomal dominant polycystic kidney disease patients. BMC Nephrol 16:86. [Europe PMC free article] [Abstract] [Google Scholar]
- Park HCKim JCho AKim DHLee YKRyu HKim HOh KHOh YKHwang YH, et al. ; KNOW-CKD Investigators Group (2020) Urinary angiotensinogen in addition to imaging classification in the prediction of renal outcome in autosomal dominant polycystic kidney disease. J Korean Med Sci 35:e165. [Europe PMC free article] [Abstract] [Google Scholar]
- Park JH, Jang HR, Lee JH, Lee JE, Huh W, Lee KB, Kwon YJ, Do JY, Kim HY, Kim YG (2015b) Comparison of intrarenal renin-angiotensin system activity in diabetic versus non-diabetic patients with overt proteinuria. Nephrology (Carlton) 20:279–285. [Abstract] [Google Scholar]
- Park S, Bivona BJ, Kobori H, Seth DM, Chappell MC, Lazartigues E, Harrison-Bernard LM (2010) Major role for ACE-independent intrarenal ANG II formation in type II diabetes. Am J Physiol Renal Physiol 298:F37–F48. [Europe PMC free article] [Abstract] [Google Scholar]
- Parving HHBrenner BMMcMurray JJde Zeeuw DHaffner SMSolomon SDChaturvedi NPersson FDesai ASNicolaides M, et al. ; ALTITUDE Investigators (2012) Cardiorenal end points in a trial of aliskiren for type 2 diabetes. N Engl J Med 367:2204–2213. [Abstract] [Google Scholar]
- Patel SN, Ali Q, Samuel P, Steckelings UM, Hussain T (2017) Angiotensin II type 2 receptor and receptor Mas are colocalized and functionally interdependent in obese Zucker rat kidney. Hypertension 70:831–838. [Europe PMC free article] [Abstract] [Google Scholar]
- Pavo NPrausmüller SSpinka GGoliasch GBartko PEWurm RArfsten HStrunk GPoglitsch MDomenig O, et al. (2021) Myocardial angiotensin metabolism in end-stage heart failure. J Am Coll Cardiol 77:1731–1743. [Abstract] [Google Scholar]
- Pentz ES, Moyano MA, Thornhill BA, Sequeira Lopez ML, Gomez RA (2004) Ablation of renin-expressing juxtaglomerular cells results in a distinct kidney phenotype. Am J Physiol Regul Integr Comp Physiol 286:R474–R483. [Abstract] [Google Scholar]
- Pessôa BS, Slump DE, Ibrahimi K, Grefhorst A, van Veghel R, Garrelds IM, Roks AJ, Kushner SA, Danser AH, van Esch JH (2015) Angiotensin II type 2 receptor- and acetylcholine-mediated relaxation: essential contribution of female sex hormones and chromosomes. Hypertension 66:396–402. [Abstract] [Google Scholar]
- Pilz BShagdarsuren EWellner MFiebeler ADechend RGratze PMeiners SFeldman DLWebb RLGarrelds IM, et al. (2005) Aliskiren, a human renin inhibitor, ameliorates cardiac and renal damage in double-transgenic rats. Hypertension 46:569–576. [Abstract] [Google Scholar]
- Pinheiro SVBFerreira AJKitten GTda Silveira KDda Silva DASantos SHSGava ECastro CHMagalhães JAda Mota RK, et al. (2009) Genetic deletion of the angiotensin-(1-7) receptor Mas leads to glomerular hyperfiltration and microalbuminuria. Kidney Int 75:1184–1193. [Abstract] [Google Scholar]
- Pohl M, Kaminski H, Castrop H, Bader M, Himmerkus N, Bleich M, Bachmann S, Theilig F (2010) Intrarenal renin angiotensin system revisited: role of megalin-dependent endocytosis along the proximal nephron. J Biol Chem 285:41935–41946. [Europe PMC free article] [Abstract] [Google Scholar]
- Ponikowski PVoors AAAnker SDBueno HCleland JGFCoats AJSFalk VGonzález-Juanatey JRHarjola V-PJankowska EA, et al. ; Authors/Task Force Members; Document Reviewers (2016) 2016 ESC Guidelines for the diagnosis and treatment of acute and chronic heart failure: the Task Force for the diagnosis and treatment of acute and chronic heart failure of the European Society of Cardiology (ESC). Developed with the special contribution of the Heart Failure Association (HFA) of the ESC. Eur J Heart Fail 18:891–975. [Abstract] [Google Scholar]
- Price DA, Porter LE, Gordon M, Fisher ND, De’Oliveira JM, Laffel LM, Passan DR, Williams GH, Hollenberg NK (1999) The paradox of the low-renin state in diabetic nephropathy. J Am Soc Nephrol 10:2382–2391. [Abstract] [Google Scholar]
- Prieto-Carrasquero MC, Harrison-Bernard LM, Kobori H, Ozawa Y, Hering-Smith KS, Hamm LL, Navar LG (2004) Enhancement of collecting duct renin in angiotensin II-dependent hypertensive rats. Hypertension 44:223–229. [Europe PMC free article] [Abstract] [Google Scholar]
- Qi Z, Hao CM, Langenbach RI, Breyer RM, Redha R, Morrow JD, Breyer MD (2002) Opposite effects of cyclooxygenase-1 and -2 activity on the pressor response to angiotensin II. J Clin Invest 110:61–69. [Europe PMC free article] [Abstract] [Google Scholar]
- Quadri S, Siragy HM (2014) Regulation of (pro)renin receptor expression in mIMCD via the GSK-3β-NFAT5-SIRT-1 signaling pathway. Am J Physiol Renal Physiol 307:F593–F600. [Europe PMC free article] [Abstract] [Google Scholar]
- Quadri S, Siragy HM (2016) (Pro)renin receptor contributes to regulation of renal epithelial sodium channel. J Hypertens 34:486–494, discussion 494. [Europe PMC free article] [Abstract] [Google Scholar]
- Rahman A, Hitomi H, Nishiyama A (2017) Cardioprotective effects of SGLT2 inhibitors are possibly associated with normalization of the circadian rhythm of blood pressure. Hypertens Res 40:535–540. [Abstract] [Google Scholar]
- Ramkumar N, Stuart D, Calquin M, Quadri S, Wang S, Van Hoek AN, Siragy HM, Ichihara A, Kohan DE (2015) Nephron-specific deletion of the prorenin receptor causes a urine concentration defect. Am J Physiol Renal Physiol 309:F48–F56. [Europe PMC free article] [Abstract] [Google Scholar]
- Ramkumar N, Stuart D, Calquin M, Wang S, Niimura F, Matsusaka T, Kohan DE (2016a) Possible role for nephron-derived angiotensinogen in angiotensin-II dependent hypertension. Physiol Rep 4:e12675. [Europe PMC free article] [Abstract] [Google Scholar]
- Ramkumar N, Stuart D, Mironova E, Abraham N, Gao Y, Wang S, Lakshmipathi J, Stockand JD, Kohan DE (2018) Collecting duct principal, but not intercalated, cell prorenin receptor regulates renal sodium and water excretion. Am J Physiol Renal Physiol 315:F607–F617. [Europe PMC free article] [Abstract] [Google Scholar]
- Ramkumar N, Stuart D, Mironova E, Bugay V, Wang S, Abraham N, Ichihara A, Stockand JD, Kohan DE (2016b) Renal tubular epithelial cell prorenin receptor regulates blood pressure and sodium transport. Am J Physiol Renal Physiol 311:F186–F194. [Europe PMC free article] [Abstract] [Google Scholar]
- Ramkumar N, Stuart D, Peterson CS, Hu C, Wheatley W, Min Cho J, Symons JD, Kohan DE (2021) Loss of soluble (pro)renin receptor attenuates angiotensin-II induced hypertension and renal injury. Circ Res 129:50–62. [Europe PMC free article] [Abstract] [Google Scholar]
- Rehman A, Leibowitz A, Yamamoto N, Rautureau Y, Paradis P, Schiffrin EL (2012) Angiotensin type 2 receptor agonist compound 21 reduces vascular injury and myocardial fibrosis in stroke-prone spontaneously hypertensive rats. Hypertension 59:291–299. [Abstract] [Google Scholar]
- Reich HN, Oudit GY, Penninger JM, Scholey JW, Herzenberg AM (2008) Decreased glomerular and tubular expression of ACE2 in patients with type 2 diabetes and kidney disease. Kidney Int 74:1610–1616. [Abstract] [Google Scholar]
- Ren LSun YLu HYe DHan LWang NDaugherty ALi FWang MSu F, et al. (2018) (Pro)renin receptor inhibition reprograms hepatic lipid metabolism and protects mice from diet-induced obesity and hepatosteatosis. Circ Res 122:730–741. [Europe PMC free article] [Abstract] [Google Scholar]
- Reyes-Martinez C, Nguyen QM, Kassan M, Gonzalez AA (2019) (Pro)renin receptor-dependent induction of profibrotic factors is mediated by COX-2/EP4/NOX-4/Smad pathway in collecting duct cells. Front Pharmacol 10:803. [Europe PMC free article] [Abstract] [Google Scholar]
- Rice GI, Thomas DA, Grant PJ, Turner AJ, Hooper NM (2004) Evaluation of angiotensin-converting enzyme (ACE), its homologue ACE2 and neprilysin in angiotensin peptide metabolism. Biochem J 383:45–51. [Europe PMC free article] [Abstract] [Google Scholar]
- Richer JDaoud HGeier PJarinova OCarson NFeberova JBen Fadel NUnrau JBareke EKhatchadourian K, et al. (2015) Resolution of refractory hypotension and anuria in a premature newborn with loss-of-function of ACE. Am J Med Genet A 167:1654–1658. [Abstract] [Google Scholar]
- Riquier-Brison ADMSipos APrókai ÁVargas SLToma LMeer EJVillanueva KGChen JCMGyarmati GYih C, et al. (2018) The macula densa prorenin receptor is essential in renin release and blood pressure control. Am J Physiol Renal Physiol 315:F521–F534. [Europe PMC free article] [Abstract] [Google Scholar]
- Rohrwasser AMorgan TDillon HFZhao LCallaway CWHillas EZhang SCheng TInagami TWard K, et al. (1999) Elements of a paracrine tubular renin-angiotensin system along the entire nephron. Hypertension 34:1265–1274. [Abstract] [Google Scholar]
- Roksnoer LCHeijnen BFNakano DPeti-Peterdi JWalsh SBGarrelds IMvan Gool JMZietse RStruijker-Boudier HAHoorn EJ, et al. (2016a) On the origin of urinary renin: a translational approach. Hypertension 67:927–933. [Europe PMC free article] [Abstract] [Google Scholar]
- Roksnoer LCvan Veghel RClahsen-van Groningen MCde Vries RGarrelds IMBhaggoe UMvan Gool JMFriesema ECLeijten FPHoorn EJ, et al. (2016b) Blood pressure-independent renoprotection in diabetic rats treated with AT1 receptor-neprilysin inhibition compared with AT1 receptor blockade alone. Clin Sci (Lond) 130:1209–1220. [Abstract] [Google Scholar]
- Roksnoer LCvan Veghel Rde Vries RGarrelds IMBhaggoe UMFriesema ECLeijten FPPoglitsch MDomenig OClahsen-van Groningen MC, et al. (2015) Optimum AT1 receptor-neprilysin inhibition has superior cardioprotective effects compared with AT1 receptor blockade alone in hypertensive rats. Kidney Int 88:109–120. [Abstract] [Google Scholar]
- Roksnoer LCvan Veghel Rvan Groningen MCde Vries RGarrelds IMBhaggoe UMvan Gool JMFriesema ECLeijten FPHoorn EJ, et al. (2016c) Blood pressure-independent renoprotection in diabetic rats treated with AT1 receptor-neprilysin inhibition compared with AT1 receptor blockade alone. Clin Sci (Lond) 130:1209–1220. [Abstract] [Google Scholar]
- Rossing P, Strand J, Avogaro A, Becka M, Kanefendt F, Otto C (2021) Effects of the chymase inhibitor fulacimstat in diabetic kidney disease-results from the CADA DIA trial. Nephrol Dial Transplant 36:2263–2273. [Abstract] [Google Scholar]
- Rüster C, Wolf G (2006) Renin-angiotensin-aldosterone system and progression of renal disease. J Am Soc Nephrol 17:2985–2991. [Abstract] [Google Scholar]
- Safari T, Nematbakhsh M, Hilliard LM, Evans RG, Denton KM (2012) Sex differences in the renal vascular response to angiotensin II involves the Mas receptor. Acta Physiol (Oxf) 206:150–156. [Abstract] [Google Scholar]
- Saito T, Urushihara M, Kotani Y, Kagami S, Kobori H (2009) Increased urinary angiotensinogen is precedent to increased urinary albumin in patients with type 1 diabetes. Am J Med Sci 338:478–480. [Europe PMC free article] [Abstract] [Google Scholar]
- Salih M, Bovée DM, Roksnoer LCW, Casteleijn NF, Bakker SJL, Gansevoort RT, Zietse R, Danser AHJ, Hoorn EJ (2017) Urinary renin-angiotensin markers in polycystic kidney disease. Am J Physiol Renal Physiol 313:F874–F881. [Abstract] [Google Scholar]
- Salinas-Parra N, Reyes-Martínez C, Prieto MC, Gonzalez AA (2017) Prostaglandin E2 induces prorenin-dependent activation of (pro)renin receptor and upregulation of cyclooxygenase-2 in collecting duct cells. Am J Med Sci 354:310–318. [Europe PMC free article] [Abstract] [Google Scholar]
- Sampaio WO, Henrique de Castro C, Santos RA, Schiffrin EL, Touyz RM (2007a) Angiotensin-(1-7) counterregulates angiotensin II signaling in human endothelial cells. Hypertension 50:1093–1098. [Abstract] [Google Scholar]
- Sampaio WO, Souza dos Santos RA, Faria-Silva R, da Mata Machado LT, Schiffrin EL, Touyz RM (2007b) Angiotensin-(1-7) through receptor Mas mediates endothelial nitric oxide synthase activation via Akt-dependent pathways. Hypertension 49:185–192. [Abstract] [Google Scholar]
- Sampson AK, Moritz KM, Denton KM (2012) Postnatal ontogeny of angiotensin receptors and ACE2 in male and female rats. Gend Med 9:21–32. [Abstract] [Google Scholar]
- Santos RA, Baracho NC (1992) Angiotensin-(1-7) is a potent antidiuretic peptide in rats. Braz J Med Biol Res 25:651–654. [Abstract] [Google Scholar]
- Santos RA, Simões e Silva AC, Magaldi AJ, Khosla MC, Cesar KR, Passaglio KT, Baracho NC (1996) Evidence for a physiological role of angiotensin-(1-7) in the control of hydroelectrolyte balance. Hypertension 27:875–884. [Abstract] [Google Scholar]
- Santos RASimoes e Silva ACMaric CSilva DMMachado RPde Buhr IHeringer-Walther SPinheiro SVLopes MTBader M, et al. (2003) Angiotensin-(1-7) is an endogenous ligand for the G protein-coupled receptor Mas. Proc Natl Acad Sci USA 100:8258–8263. [Europe PMC free article] [Abstract] [Google Scholar]
- Satirapoj B, Pooluea P, Nata N, Supasyndh O (2019) Urinary biomarkers of tubular injury to predict renal progression and end stage renal disease in type 2 diabetes mellitus with advanced nephropathy: a prospective cohort study. J Diabetes Complications 33:675–681. [Abstract] [Google Scholar]
- Satirapoj B, Siritaweesuk N, Supasyndh O (2014) Urinary angiotensinogen as a potential biomarker of diabetic nephropathy. Clin Kidney J 7:354–360. [Europe PMC free article] [Abstract] [Google Scholar]
- Satou R, Cypress MW, Woods TC, Katsurada A, Dugas CM, Fonseca VA, Navar LG (2020) Blockade of sodium-glucose cotransporter 2 suppresses high glucose-induced angiotensinogen augmentation in renal proximal tubular cells. Am J Physiol Renal Physiol 318:F67–F75. [Europe PMC free article] [Abstract] [Google Scholar]
- Schalekamp MA, Danser AH (2006a) Angiotensin II production and distribution in the kidney--II. Model-based analysis of experimental data. Kidney Int 69:1553–1557. [Abstract] [Google Scholar]
- Schalekamp MA, Danser AH (2006b) Angiotensin II production and distribution in the kidney: I. A kinetic model. Kidney Int 69:1543–1552. [Abstract] [Google Scholar]
- Schutgens FRookmaaker MBMargaritis TRios AAmmerlaan CJansen JGijzen LVormann MVonk AViveen M, et al. (2019) Tubuloids derived from human adult kidney and urine for personalized disease modeling. Nat Biotechnol 37:303–313. [Abstract] [Google Scholar]
- Schweda F, Klar J, Narumiya S, Nüsing RM, Kurtz A (2004) Stimulation of renin release by prostaglandin E2 is mediated by EP2 and EP4 receptors in mouse kidneys. Am J Physiol Renal Physiol 287:F427–F433. [Abstract] [Google Scholar]
- Sechi LA, Grady EF, Griffin CA, Kalinyak JE, Schambelan M (1992) Distribution of angiotensin II receptor subtypes in rat and human kidney. Am J Physiol 262:F236–F240. [Abstract] [Google Scholar]
- Sequeira-Lopez ML, Lin EE, Li M, Hu Y, Sigmund CD, Gomez RA (2015) The earliest metanephric arteriolar progenitors and their role in kidney vascular development. Am J Physiol Regul Integr Comp Physiol 308:R138–R149. [Europe PMC free article] [Abstract] [Google Scholar]
- Sequeira López ML, Pentz ES, Nomasa T, Smithies O, Gomez RA (2004) Renin cells are precursors for multiple cell types that switch to the renin phenotype when homeostasis is threatened. Dev Cell 6:719–728. [Abstract] [Google Scholar]
- Sequeira-Lopez MLS, Gomez RA (2021) Renin cells, the kidney, and hypertension. Circ Res 128:887–907. [Europe PMC free article] [Abstract] [Google Scholar]
- Serfozo PWysocki JGulua GSchulze AYe MLiu PJin JBader MMyöhänen TGarcía-Horsman JA, et al. (2020) Ang II (angiotensin II) conversion to angiotensin-(1-7) in the circulation is POP (prolyloligopeptidase)-dependent and ACE2 (angiotensin-converting enzyme 2)-independent. Hypertension 75:173–182. [Europe PMC free article] [Abstract] [Google Scholar]
- Shankar ASDu ZMora HTvan den Bosch TPPKorevaar SSVan den Berg-Garrelds IMBindels ELopez-Iglesias CClahsen-van Groningen MCGribnau J, et al. (2021) Human kidney organoids produce functional renin. Kidney Int 99:134–147. [Abstract] [Google Scholar]
- Shin SJChung SKim SJLee EMYoo YHKim JWAhn YBKim ESMoon SDKim MJ, et al. (2016) Effect of sodium-glucose co-transporter 2 inhibitor, dapagliflozin, on renal renin-angiotensin system in an animal model of type 2 diabetes. PLoS One 11:e0165703. [Europe PMC free article] [Abstract] [Google Scholar]
- Shirazi M, Wysocki J, Ye M, Haney C, Zhao M, Kanwar YS, Zhang ZJ, Batlle D (2019) A novel angiotensin-converting enzyme 2 truncate markedly improves ischemic AKI (Abstract) in ASN Kidney Week; 2019 Nov 5–10; Washington, DC. American Society of Nephrology, Washington, DC. [Google Scholar]
- Shulla A, Heald-Sargent T, Subramanya G, Zhao J, Perlman S, Gallagher T (2011) A transmembrane serine protease is linked to the severe acute respiratory syndrome coronavirus receptor and activates virus entry. J Virol 85:873–882. [Europe PMC free article] [Abstract] [Google Scholar]
- Simões e Silva AC, Bello AP, Baracho NC, Khosla MC, Santos RA (1998) Diuresis and natriuresis produced by long term administration of a selective angiotensin-(1-7) antagonist in normotensive and hypertensive rats. Regul Pept 74:177–184. [Abstract] [Google Scholar]
- Singh R, Singh AK, Leehey DJ (2005) A novel mechanism for angiotensin II formation in streptozotocin-diabetic rat glomeruli. Am J Physiol Renal Physiol 288:F1183–F1190. [Abstract] [Google Scholar]
- Siragy HM, Inagami T, Ichiki T, Carey RM (1999) Sustained hypersensitivity to angiotensin II and its mechanism in mice lacking the subtype-2 (AT2) angiotensin receptor. Proc Natl Acad Sci USA 96:6506–6510. [Europe PMC free article] [Abstract] [Google Scholar]
- Song R, Preston G, Ichihara A, Yosypiv IV (2013) Deletion of the prorenin receptor from the ureteric bud causes renal hypodysplasia. PLoS One 8:e63835. [Europe PMC free article] [Abstract] [Google Scholar]
- Song R, Preston G, Kidd L, Bushnell D, Sims-Lucas S, Bates CM, Yosypiv IV (2016) Prorenin receptor is critical for nephron progenitors. Dev Biol 409:382–391. [Europe PMC free article] [Abstract] [Google Scholar]
- Stegbauer J, Chen D, Herrera M, Sparks MA, Yang T, Königshausen E, Gurley SB, Coffman TM (2017) Resistance to hypertension mediated by intercalated cells of the collecting duct. JCI Insight 2:e92720. [Europe PMC free article] [Abstract] [Google Scholar]
- Su Z, Zimpelmann J, Burns KD (2006) Angiotensin-(1-7) inhibits angiotensin II-stimulated phosphorylation of MAP kinases in proximal tubular cells. Kidney Int 69:2212–2218. [Abstract] [Google Scholar]
- Sullivan JC, Bhatia K, Yamamoto T, Elmarakby AA (2010) Angiotensin (1-7) receptor antagonism equalizes angiotensin II-induced hypertension in male and female spontaneously hypertensive rats. Hypertension 56:658–666. [Europe PMC free article] [Abstract] [Google Scholar]
- Sun Y, Bovée DM, Danser AHJ (2019) Tubular (pro)renin release. Hypertension 74:26–28. [Abstract] [Google Scholar]
- Sun Y, Danser AHJ, Lu X (2017) (Pro)renin receptor as a therapeutic target for the treatment of cardiovascular diseases? Pharmacol Res 125 (Pt A):48–56. [Abstract] [Google Scholar]
- Sun Y, Goes Martini A, Janssen MJ, Garrelds IM, Masereeuw R, Lu X, Danser AHJ (2020a) Megalin: a novel endocytic receptor for prorenin and renin. Hypertension 75:1242–1250. [Abstract] [Google Scholar]
- Sun Y, Lu X, Danser AHJ (2020b) Megalin: a novel determinant of renin-angiotensin system activity in the kidney? Curr Hypertens Rep 22:30. [Europe PMC free article] [Abstract] [Google Scholar]
- Takamatsu M, Urushihara M, Kondo S, Shimizu M, Morioka T, Oite T, Kobori H, Kagami S (2008) Glomerular angiotensinogen protein is enhanced in pediatric IgA nephropathy. Pediatr Nephrol 23:1257–1267. [Europe PMC free article] [Abstract] [Google Scholar]
- Takasato MEr PXChiu HSMaier BBaillie GJFerguson CParton RGWolvetang EJRoost MSChuva de Sousa Lopes SM, et al. (2015) Kidney organoids from human iPS cells contain multiple lineages and model human nephrogenesis. Nature 526:564–568. [Abstract] [Google Scholar]
- Takeshige YFujisawa YRahman AKittikulsuth WNakano DMori HMasaki TOhmori KKohno MOgata H, et al. (2016) A sodium-glucose co-transporter 2 inhibitor empagliflozin prevents abnormality of circadian rhythm of blood pressure in salt-treated obese rats. Hypertens Res 39:415–422. [Abstract] [Google Scholar]
- Tang JWysocki JYe MVallés PGRein JShirazi MBader MGomez RASequeira-Lopez MSAfkarian M, et al. (2019) Urinary renin in patients and mice with diabetic kidney disease. Hypertension 74:83–94. [Europe PMC free article] [Abstract] [Google Scholar]
- Tang L, Loutzenhiser K, Loutzenhiser R (2000) Biphasic actions of prostaglandin E(2) on the renal afferent arteriole : role of EP(3) and EP(4) receptors. Circ Res 86:663–670. [Abstract] [Google Scholar]
- Tang Z, Wang Y, Tao L, Guo Y, Zheng Y, Zheng D (2018) The elevated levels of urinary angiotensinogen are correlated with the severity of idiopathic membranous nephropathy. BMC Nephrol 19:357. [Europe PMC free article] [Abstract] [Google Scholar]
- te Riet Lvan den Heuvel MPeutz-Kootstra CJvan Esch JHvan Veghel RGarrelds IMMusterd-Bhaggoe UBouhuizen AMLeijten FPDanser AH, et al. (2014) Deterioration of kidney function by the (pro)renin receptor blocker handle region peptide in aliskiren-treated diabetic transgenic (mRen2)27 rats. Am J Physiol Renal Physiol 306:F1179–F1189. [Abstract] [Google Scholar]
- Terami T, Wada J, Inoue K, Nakatsuka A, Ogawa D, Teshigawara S, Murakami K, Katayama A, Eguchi J, Makino H (2013) Urinary angiotensinogen is a marker for tubular injuries in patients with type 2 diabetes. Int J Nephrol Renovasc Dis 6:233–240. [Europe PMC free article] [Abstract] [Google Scholar]
- Thomopoulos C, Parati G, Zanchetti A (2017) Effects of blood-pressure-lowering treatment on outcome incidence in hypertension: 10 - should blood pressure management differ in hypertensive patients with and without diabetes mellitus? Overview and meta-analyses of randomized trials. J Hypertens 35:922–944. [Abstract] [Google Scholar]
- Tikellis C, Cooper ME, Bialkowski K, Johnston CI, Burns WC, Lew RA, Smith AI, Thomas MC (2006) Developmental expression of ACE2 in the SHR kidney: a role in hypertension? Kidney Int 70:34–41. [Abstract] [Google Scholar]
- Tipnis SR, Hooper NM, Hyde R, Karran E, Christie G, Turner AJ (2000) A human homolog of angiotensin-converting enzyme. Cloning and functional expression as a captopril-insensitive carboxypeptidase. J Biol Chem 275:33238–33243. [Abstract] [Google Scholar]
- Tocci G, Paneni F, Palano F, Sciarretta S, Ferrucci A, Kurtz T, Mancia G, Volpe M (2011) Angiotensin-converting enzyme inhibitors, angiotensin II receptor blockers and diabetes: a meta-analysis of placebo-controlled clinical trials. Am J Hypertens 24:582–590. [Abstract] [Google Scholar]
- Tom B, de Vries R, Saxena PR, Danser AH (2001) Bradykinin potentiation by angiotensin-(1-7) and ACE inhibitors correlates with ACE C- and N-domain blockade. Hypertension 38:95–99. [Abstract] [Google Scholar]
- Tornling GBatta RPorter JCWilliams BBengtsson TParmar KKashiva RHallberg ACohrt AKWestergaard K, et al. (2021) Seven days treatment with the angiotensin II type 2 receptor agonist C21 in hospitalized COVID-19 patients; a placebo-controlled randomised multi-centre double-blind phase 2 trial. EClinicalMedicine 41:101152. [Europe PMC free article] [Abstract] [Google Scholar]
- Traynor TR, Smart A, Briggs JP, Schnermann J (1999) Inhibition of macula densa-stimulated renin secretion by pharmacological blockade of cyclooxygenase-2. Am J Physiol 277:F706–F710. [Abstract] [Google Scholar]
- Trepiccione FGerber SDGrahammer FLópez-Cayuqueo KIBaudrie VPăunescu TGCapen DEPicard NAlexander RTHuber TB, et al. (2016) Renal Atp6ap2/(pro)renin receptor is required for normal vacuolar H+-ATPase function but not for the renin-angiotensin system. J Am Soc Nephrol 27:3320–3330. [Europe PMC free article] [Abstract] [Google Scholar]
- Tufro-McReddie A, Johns DW, Geary KM, Dagli H, Everett AD, Chevalier RL, Carey RM, Gomez RA (1994) Angiotensin II type 1 receptor: role in renal growth and gene expression during normal development. Am J Physiol 266:F911–F918. [Abstract] [Google Scholar]
- Turner AJ, Hooper NM (2004) Angiotensin-converting enzyme 2, in Handbook of Proteolytic Enzymes, 2nd ed (Barrett AJ, Rawlings ND, Woessner JF, eds) pp 349–351, Academic Press, Cambridge, MA. [Google Scholar]
- Uhlenius N, Miettinen A, Vuolteenaho O, Tikkanen I (2002) Renoprotective mechanisms of angiotensin II antagonism in experimental chronic renal failure. Kidney Blood Press Res 25:71–79. [Abstract] [Google Scholar]
- Uijl EMirabito Colafella KMSun YRen Lvan Veghel RGarrelds IMde Vries RPoglitsch MZlatev IKim JB, et al. (2019) Strong and sustained antihypertensive effect of small interfering RNA targeting liver angiotensinogen. Hypertension 73:1249–1257. [Abstract] [Google Scholar]
- Uijl ERen LMirabito Colafella KMvan Veghel RGarrelds IMDomenig OPoglitsch MZlatev IKim JBHuang S, et al. (2021) No evidence for brain renin-angiotensin system activation during DOCA-salt hypertension. Clin Sci (Lond) 135:259–274. [Abstract] [Google Scholar]
- Uijl E’t Hart DCRoksnoer LCWGroningen MCCvan Veghel RGarrelds IMde Vries Rvan der Vlag JZietse RNijenhuis T, et al. (2020) Angiotensin-neprilysin inhibition confers renoprotection in rats with diabetes and hypertension by limiting podocyte injury. J Hypertens 38:755–764. [Abstract] [Google Scholar]
- Urata H, Kinoshita A, Misono KS, Bumpus FM, Husain A (1990) Identification of a highly specific chymase as the major angiotensin II-forming enzyme in the human heart. J Biol Chem 265:22348–22357. [Abstract] [Google Scholar]
- Urushihara M, Kondo S, Kagami S, Kobori H (2010) Urinary angiotensinogen accurately reflects intrarenal renin-angiotensin system activity. Am J Nephrol 31:318–325. [Europe PMC free article] [Abstract] [Google Scholar]
- Urushihara M, Nagai T, Kinoshita Y, Nishiyama S, Suga K, Ozaki N, Jamba A, Kondo S, Kobori H, Kagami S (2015) Changes in urinary angiotensinogen posttreatment in pediatric IgA nephropathy patients. Pediatr Nephrol 30:975–982. [Europe PMC free article] [Abstract] [Google Scholar]
- van den Heuvel M, Batenburg WW, Jainandunsing S, Garrelds IM, van Gool JM, Feelders RA, van den Meiracker AH, Danser AH (2011) Urinary renin, but not angiotensinogen or aldosterone, reflects the renal renin-angiotensin-aldosterone system activity and the efficacy of renin-angiotensin-aldosterone system blockade in the kidney. J Hypertens 29:2147–2155. [Abstract] [Google Scholar]
- van der Heijden BJ, Carlus C, Narcy F, Bavoux F, Delezoide AL, Gubler MC (1994) Persistent anuria, neonatal death, and renal microcystic lesions after prenatal exposure to indomethacin. Am J Obstet Gynecol 171:617–623. [Abstract] [Google Scholar]
- van Esch JH, Moltzer E, van Veghel R, Garrelds IM, Leijten F, Bouhuizen AM, Danser AH (2010a) Beneficial cardiac effects of the renin inhibitor aliskiren in spontaneously hypertensive rats. J Hypertens 28:2145–2155. [Abstract] [Google Scholar]
- van Esch JH, Oosterveer CR, Batenburg WW, van Veghel R, Jan Danser AH (2008) Effects of angiotensin II and its metabolites in the rat coronary vascular bed: is angiotensin III the preferred ligand of the angiotensin AT2 receptor? Eur J Pharmacol 588:286–293. [Abstract] [Google Scholar]
- van Esch JH, Tom B, Dive V, Batenburg WW, Georgiadis D, Yiotakis A, van Gool JM, de Bruijn RJ, de Vries R, Danser AH (2005) Selective angiotensin-converting enzyme C-domain inhibition is sufficient to prevent angiotensin I-induced vasoconstriction. Hypertension 45:120–125. [Abstract] [Google Scholar]
- van Esch JHMGembardt FSterner-Kock AHeringer-Walther SLe THLassner DStijnen TCoffman TMSchultheiss H-PDanser AHJ, et al. (2010b) Cardiac phenotype and angiotensin II levels in AT1a, AT1b, and AT2 receptor single, double, and triple knockouts. Cardiovasc Res 86:401–409. [Europe PMC free article] [Abstract] [Google Scholar]
- van Kats JP, de Lannoy LM, Danser AH, van Meegen JR, Verdouw PD, Schalekamp MA (1997) Angiotensin II type 1 (AT1) receptor-mediated accumulation of angiotensin II in tissues and its intracellular half-life in vivo. Hypertension 30:42–49. [Abstract] [Google Scholar]
- van Kats JP, Schalekamp MA, Verdouw PD, Duncker DJ, Danser AH (2001a) Intrarenal angiotensin II: interstitial and cellular levels and site of production. Kidney Int 60:2311–2317. [Abstract] [Google Scholar]
- van Kats JP, van Meegen JR, Verdouw PD, Duncker DJ, Schalekamp MA, Danser AH (2001b) Subcellular localization of angiotensin II in kidney and adrenal. J Hypertens 19:583–589. [Abstract] [Google Scholar]
- Vázquez E, Coronel I, Bautista R, Romo E, Villalón CM, Avila-Casado MC, Soto V, Escalante B (2005) Angiotensin II-dependent induction of AT(2) receptor expression after renal ablation. Am J Physiol Renal Physiol 288:F207–F213. [Abstract] [Google Scholar]
- Verdonk K, Danser AH, van Esch JH (2012a) Angiotensin II type 2 receptor agonists: where should they be applied? Expert Opin Investig Drugs 21:501–513. [Abstract] [Google Scholar]
- Verdonk K, Durik M, Abd-Alla N, Batenburg WW, van den Bogaerdt AJ, van Veghel R, Roks AJ, Danser AH, van Esch JH (2012b) Compound 21 induces vasorelaxation via an endothelium- and angiotensin II type 2 receptor-independent mechanism. Hypertension 60:722–729. [Abstract] [Google Scholar]
- Vermes E, Ducharme A, Bourassa MG, Lessard M, White M, Tardif J-C; Studies Of Left Ventricular Dysfunction (2003) Enalapril reduces the incidence of diabetes in patients with chronic heart failure: insight from the Studies Of Left Ventricular Dysfunction (SOLVD). Circulation 107:1291–1296. [Abstract] [Google Scholar]
- Vickers CHales PKaushik VDick LGavin JTang JGodbout KParsons TBaronas EHsieh F, et al. (2002) Hydrolysis of biological peptides by human angiotensin-converting enzyme-related carboxypeptidase. J Biol Chem 277:14838–14843. [Abstract] [Google Scholar]
- Viegas VU, Liu ZZ, Nikitina T, Perlewitz A, Zavaritskaya O, Schlichting J, Persson PB, Regitz-Zagrosek V, Patzak A, Sendeski MM (2012) Angiotensin II type 2 receptor mediates sex differences in mice renal interlobar arteries response to angiotensin II. J Hypertens 30:1791–1798. [Abstract] [Google Scholar]
- Violin JD, Soergel DG, Boerrigter G, Burnett JC Jr, Lark MW (2013) GPCR biased ligands as novel heart failure therapeutics. Trends Cardiovasc Med 23:242–249. [Abstract] [Google Scholar]
- Vogt L, de Zeeuw D, Woittiez AJ, Navis G (2009) Selective cyclooxygenase-2 (COX-2) inhibition reduces proteinuria in renal patients. Nephrol Dial Transplant 24:1182–1189. [Abstract] [Google Scholar]
- Voors AAGori MLiu LCClaggett BZile MRPieske BMcMurray JJPacker MShi VLefkowitz MP, et al. ; PARAMOUNT Investigators (2015) Renal effects of the angiotensin receptor neprilysin inhibitor LCZ696 in patients with heart failure and preserved ejection fraction. Eur J Heart Fail 17:510–517. [Abstract] [Google Scholar]
- Wagner C, de Wit C, Kurtz L, Grünberger C, Kurtz A, Schweda F (2007) Connexin40 is essential for the pressure control of renin synthesis and secretion. Circ Res 100:556–563. [Abstract] [Google Scholar]
- Wan N, Rahman A, Hitomi H, Nishiyama A (2018) The effects of sodium-glucose cotransporter 2 inhibitors on sympathetic nervous activity. Front Endocrinol (Lausanne) 9:421. [Europe PMC free article] [Abstract] [Google Scholar]
- Wan Y, Shang J, Graham R, Baric RS, Li F (2020) Receptor recognition by the novel coronavirus from Wuhan: an analysis based on decade-long structural studies of SARS coronavirus. J Virol 94:e00127–20. [Europe PMC free article] [Abstract] [Google Scholar]
- Wang F, Chen Y, Zou CJ, Luo R, Yang T (2021) Mutagenesis of the cleavage site of pro renin receptor abrogates angiotensin II-induced hypertension in mice. Hypertension 78:115–127. [Europe PMC free article] [Abstract] [Google Scholar]
- Wang FLu XPeng KFang HZhou LSu JNau AYang KTIchihara ALu A, et al. (2016) Antidiuretic action of collecting duct (pro)renin receptor downstream of vasopressin and PGE2 receptor EP4. J Am Soc Nephrol 27:3022–3034. [Europe PMC free article] [Abstract] [Google Scholar]
- Wang F, Lu X, Peng K, Zhou L, Li C, Wang W, Yu X, Kohan DE, Zhu SF, Yang T (2014) COX-2 mediates angiotensin II-induced (pro)renin receptor expression in the rat renal medulla. Am J Physiol Renal Physiol 307:F25–F32. [Europe PMC free article] [Abstract] [Google Scholar]
- Wang F, Luo R, Peng K, Liu X, Xu C, Lu X, Soodvilai S, Yang T (2020) Soluble (pro)renin receptor regulation of ENaC involved in aldosterone signaling in cultured collecting duct cells. Am J Physiol Renal Physiol 318:F817–F825. [Europe PMC free article] [Abstract] [Google Scholar]
- Wang FXu CLuo RPeng KRamkumar NXie SLu XZhao LZuo CJKohan DE, et al. (2019) Site-1 protease-derived soluble (pro)renin receptor targets vasopressin receptor 2 to enhance urine concentrating capability. JCI Insight 4:e124174. [Europe PMC free article] [Abstract] [Google Scholar]
- Wang G, Lai FM-M, Kwan BC, Lai K-B, Chow K-M, Li PK, Szeto C-C (2011) Expression of ACE and ACE2 in patients with hypertensive nephrosclerosis. Kidney Blood Press Res 34:141–149. [Abstract] [Google Scholar]
- Wang J, Shibayama Y, Kobori H, Liu Y, Kobara H, Masaki T, Wang Z, Nishiyama A (2017a) High glucose augments angiotensinogen in human renal proximal tubular cells through hepatocyte nuclear factor-5. PLoS One 12:e0185600. [Europe PMC free article] [Abstract] [Google Scholar]
- Wang XXLevi JLuo YMyakala KHerman-Edelstein MQiu LWang DPeng YGrenz ALucia S, et al. (2017b) SGLT2 protein expression is increased in human diabetic nephropathy: SGLT2 protein inhibition decreases renal lipid accumulation, inflammation, and the development of nephropathy in diabetic mice. J Biol Chem 292:5335–5348. [Europe PMC free article] [Abstract] [Google Scholar]
- Wang Y, Huang J, Liu X, Niu Y, Zhao L, Yu Y, Zhou L, Lu L, Yu C (2017c) β-Arrestin-biased AT1R stimulation promotes extracellular matrix synthesis in renal fibrosis. Am J Physiol Renal Physiol 313:F1–F8. [Abstract] [Google Scholar]
- Watanabe H, Martini AG, Brown EA, Liang X, Medrano S, Goto S, Narita I, Arend LJ, Sequeira-Lopez MLS, Gomez RA (2021) Inhibition of the renin-angiotensin system causes concentric hypertrophy of renal arterioles in mice and humans. JCI Insight 6:e154337. [Europe PMC free article] [Abstract] [Google Scholar]
- Welch WJ, Patel K, Modlinger P, Mendonca M, Kawada N, Dennehy K, Aslam S, Wilcox CS (2007) Roles of vasoconstrictor prostaglandins, COX-1 and -2, and AT1, AT2, and TP receptors in a rat model of early 2K,1C hypertension. Am J Physiol Heart Circ Physiol 293:H2644–H2649. [Abstract] [Google Scholar]
- Wilson BA, Nautiyal M, Gwathmey TM, Rose JC, Chappell MC (2016) Evidence for a mitochondrial angiotensin-(1-7) system in the kidney. Am J Physiol Renal Physiol 310:F637–F645. [Europe PMC free article] [Abstract] [Google Scholar]
- Wong DW, Oudit GY, Reich H, Kassiri Z, Zhou J, Liu QC, Backx PH, Penninger JM, Herzenberg AM, Scholey JW (2007) Loss of angiotensin-converting enzyme-2 (Ace2) accelerates diabetic kidney injury. Am J Pathol 171:438–451. [Europe PMC free article] [Abstract] [Google Scholar]
- Woods TC, Satou R, Miyata K, Katsurada A, Dugas CM, Klingenberg NC, Fonseca VA, Navar LG (2019) Canagliflozin prevents intrarenal angiotensinogen augmentation and mitigates kidney injury and hypertension in mouse model of type 2 diabetes mellitus. Am J Nephrol 49:331–342. [Europe PMC free article] [Abstract] [Google Scholar]
- Wu HY, Huang JW, Lin HJ, Liao WC, Peng YS, Hung KY, Wu KD, Tu YK, Chien KL (2013) Comparative effectiveness of renin-angiotensin system blockers and other antihypertensive drugs in patients with diabetes: systematic review and bayesian network meta-analysis. BMJ 347:f6008. [Europe PMC free article] [Abstract] [Google Scholar]
- Wysocki J, Garcia-Halpin L, Ye M, Maier C, Sowers K, Burns KD, Batlle D (2013) Regulation of urinary ACE2 in diabetic mice. Am J Physiol Renal Physiol 305:F600–F611. [Europe PMC free article] [Abstract] [Google Scholar]
- Wysocki J, Goodling A, Burgaya M, Whitlock K, Ruzinski J, Batlle D, Afkarian M (2017a) Urine RAS components in mice and people with type 1 diabetes and chronic kidney disease. Am J Physiol Renal Physiol 313:F487–F494. [Europe PMC free article] [Abstract] [Google Scholar]
- Wysocki JOrtiz-Melo DIMattocks NKXu KPrescott JEvora KYe MSparks MAHaque SKBatlle D, et al. (2014) ACE2 deficiency increases NADPH-mediated oxidative stress in the kidney. Physiol Rep 2:e00264. [Europe PMC free article] [Abstract] [Google Scholar]
- Wysocki J, Schulze A, Batlle D (2019) Novel variants of angiotensin converting enzyme-2 of shorter molecular size to target the kidney renin angiotensin system. Biomolecules 9:886. [Europe PMC free article] [Abstract] [Google Scholar]
- Wysocki J, Ye M, Batlle D (2015) Plasma and kidney angiotensin peptides: importance of the aminopeptidase A/angiotensin III axis. Am J Hypertens 28:1418–1426. [Europe PMC free article] [Abstract] [Google Scholar]
- Wysocki J, Ye M, Hassler L, Gupta AK, Wang Y, Nicoleascu V, Randall G, Wertheim JA, Batlle D (2021) A novel soluble ACE2 Variant with prolonged duration of action neutralizes SARS-CoV-2 infection in human kidney organoids. J Am Soc Nephrol 32:795–803. [Europe PMC free article] [Abstract] [Google Scholar]
- Wysocki J, Ye M, Khattab AM, Fogo A, Martin A, David NV, Kanwar Y, Osborn M, Batlle D (2017b) Angiotensin-converting enzyme 2 amplification limited to the circulation does not protect mice from development of diabetic nephropathy. Kidney Int 91:1336–1346. [Europe PMC free article] [Abstract] [Google Scholar]
- Wysocki JYe MRodriguez EGonzález-Pacheco FRBarrios CEvora KSchuster MLoibner HBrosnihan KBFerrario CM, et al. (2010) Targeting the degradation of angiotensin II with recombinant angiotensin-converting enzyme 2: prevention of angiotensin II-dependent hypertension. Hypertension 55:90–98. [Europe PMC free article] [Abstract] [Google Scholar]
- Xu PCosta-Goncalves ACTodiras MRabelo LASampaio WOMoura MMSantos SSLuft FCBader MGross V, et al. (2008) Endothelial dysfunction and elevated blood pressure in MAS gene-deleted mice. Hypertension 51:574–580. [Abstract] [Google Scholar]
- Xu ZG, Lanting L, Vaziri ND, Li Z, Sepassi L, Rodriguez-Iturbe B, Natarajan R (2005) Upregulation of angiotensin II type 1 receptor, inflammatory mediators, and enzymes of arachidonate metabolism in obese Zucker rat kidney: reversal by angiotensin II type 1 receptor blockade. Circulation 111:1962–1969. [Abstract] [Google Scholar]
- Yamamoto T, Nakagawa T, Suzuki H, Ohashi N, Fukasawa H, Fujigaki Y, Kato A, Nakamura Y, Suzuki F, Hishida A (2007) Urinary angiotensinogen as a marker of intrarenal angiotensin II activity associated with deterioration of renal function in patients with chronic kidney disease. J Am Soc Nephrol 18:1558–1565. [Abstract] [Google Scholar]
- Yang J, Chen C, Ren H, Han Y, He D, Zhou L, Hopfer U, Jose PA, Zeng C (2012a) Angiotensin II AT(2) receptor decreases AT(1) receptor expression and function via nitric oxide/cGMP/Sp1 in renal proximal tubule cells from Wistar-Kyoto rats. J Hypertens 30:1176–1184. [Europe PMC free article] [Abstract] [Google Scholar]
- Yang T, Singh I, Pham H, Sun D, Smart A, Schnermann JB, Briggs JP (1998) Regulation of cyclooxygenase expression in the kidney by dietary salt intake. Am J Physiol 274:F481–F489. [Abstract] [Google Scholar]
- Yang XChen CTian JZha YXiong YSun ZChen PLi JYang TMa C, et al. (2015) Urinary angiotensinogen level predicts AKI in acute decompensated heart failure: a prospective, two-stage study. J Am Soc Nephrol 26:2032–2041. [Europe PMC free article] [Abstract] [Google Scholar]
- Yang XH, Wang YH, Wang JJ, Liu YC, Deng W, Qin C, Gao JL, Zhang LY (2012b) Role of angiotensin-converting enzyme (ACE and ACE2) imbalance on tourniquet-induced remote kidney injury in a mouse hindlimb ischemia-reperfusion model. Peptides 36:60–70. [Abstract] [Google Scholar]
- Yasui A, Lee G, Hirase T, Kaneko T, Kaspers S, von Eynatten M, Okamura T (2018) Empagliflozin induces transient diuresis without changing long-term overall fluid balance in Japanese patients with type 2 diabetes. Diabetes Ther 9:863–871. [Europe PMC free article] [Abstract] [Google Scholar]
- Ye FWang YWu CHowatt DAWu CHBalakrishnan AMullick AEGraham MJDanser AHJWang J, et al. (2019) Angiotensinogen and megalin interactions contribute to atherosclerosis-brief report. Arterioscler Thromb Vasc Biol 39:150–155. [Europe PMC free article] [Abstract] [Google Scholar]
- Ye M, Wysocki J, Gonzalez-Pacheco FR, Salem M, Evora K, Garcia-Halpin L, Poglitsch M, Schuster M, Batlle D (2012) Murine recombinant angiotensin-converting enzyme 2: effect on angiotensin II-dependent hypertension and distinctive angiotensin-converting enzyme 2 inhibitor characteristics on rodent and human angiotensin-converting enzyme 2. Hypertension 60:730–740. [Europe PMC free article] [Abstract] [Google Scholar]
- Ye M, Wysocki J, Naaz P, Salabat MR, LaPointe MS, Batlle D (2004) Increased ACE 2 and decreased ACE protein in renal tubules from diabetic mice: a renoprotective combination? Hypertension 43:1120–1125. [Abstract] [Google Scholar]
- Ye M, Wysocki J, William J, Soler MJ, Cokic I, Batlle D (2006) Glomerular localization and expression of angiotensin-converting enzyme 2 and angiotensin-converting enzyme: implications for albuminuria in diabetes. J Am Soc Nephrol 17:3067–3075. [Abstract] [Google Scholar]
- Yokota RRonchi FAFernandes FBJara ZPRosa RMLeite APOFiorino PFarah Vdo Nascimento NRFFonteles MC, et al. (2018) Intra-renal angiotensin levels are increased in high-fructose fed rats in the extracorporeal renal perfusion model. Front Physiol 9:1433. [Europe PMC free article] [Abstract] [Google Scholar]
- Yoshikawa A, Aizaki Y, Kusano K, Kishi F, Susumu T, Iida S, Ishiura S, Nishimura S, Shichiri M, Senbonmatsu T (2011) The (pro)renin receptor is cleaved by ADAM19 in the Golgi leading to its secretion into extracellular space. Hypertens Res 34:599–605. [Abstract] [Google Scholar]
- Yoshimoto T, Furuki T, Kobori H, Miyakawa M, Imachi H, Murao K, Nishiyama A (2017) Effects of sodium-glucose cotransporter 2 inhibitors on urinary excretion of intact and total angiotensinogen in patients with type 2 diabetes. J Investig Med 65:1057–1061. [Europe PMC free article] [Abstract] [Google Scholar]
- Yosypiv IV (2017) Prorenin receptor in kidney development. Pediatr Nephrol 32:383–392. [Abstract] [Google Scholar]
- Young D, Waitches G, Birchmeier C, Fasano O, Wigler M (1986) Isolation and characterization of a new cellular oncogene encoding a protein with multiple potential transmembrane domains. Cell 45:711–719. [Abstract] [Google Scholar]
- Zelniker TAWiviott SDRaz IIm KGoodrich ELBonaca MPMosenzon OKato ETCahn AFurtado RHM, et al. (2019) SGLT2 inhibitors for primary and secondary prevention of cardiovascular and renal outcomes in type 2 diabetes: a systematic review and meta-analysis of cardiovascular outcome trials. Lancet 393:31–39. [Abstract] [Google Scholar]
- Zhang MZ, Wang S, Wang Y, Zhang Y, Ming Hao C, Harris RC (2018) Renal medullary interstitial COX-2 (cyclooxygenase-2) is essential in preventing salt-sensitive hypertension and maintaining renal inner medulla/papilla structural integrity. Hypertension 72:1172–1179. [Europe PMC free article] [Abstract] [Google Scholar]
- Zhang MZ, Yao B, Cheng HF, Wang SW, Inagami T, Harris RC (2006) Renal cortical cyclooxygenase 2 expression is differentially regulated by angiotensin II AT(1) and AT(2) receptors. Proc Natl Acad Sci USA 103:16045–16050. [Europe PMC free article] [Abstract] [Google Scholar]
- Zhang XY, Ding XQ, Lv WL, Teng J, Zhong YH (2013) ELISA examining urinary angiotensinogen as a potential indicator of intrarenal renin-angiotensin system (RAS) activity: a clinical study of 128 chronic kidney disease patients. Mol Biol Rep 40:5817–5824. [Abstract] [Google Scholar]
- Zhang Z, Jiang SM, Ma YP, Dai PL, Wang YN, Zou GM, Gao HM, Yang Y, Li WG (2019) Expression of the intrarenal angiotensin receptor and the role of renin-angiotensin system inhibitors in IgA nephropathy. Mol Cell Biochem 453:103–110. [Abstract] [Google Scholar]
- Zhong J-C, Huang D-Y, Yang Y-M, Li Y-F, Liu G-F, Song X-H, Du K (2004) Upregulation of angiotensin-converting enzyme 2 by all-trans retinoic acid in spontaneously hypertensive rats. Hypertension 44:907–912. [Abstract] [Google Scholar]
- Zhuang Z, Bai Q, A L, Liang Y, Zheng D, Wang Y (2015) Increased urinary angiotensinogen precedes the onset of albuminuria in normotensive type 2 diabetic patients. Int J Clin Exp Pathol 8:11464–11469. [Europe PMC free article] [Abstract] [Google Scholar]
- Zhuo J, Alcorn D, Allen AM, Mendelsohn FA (1992) High resolution localization of angiotensin II receptors in rat renal medulla. Kidney Int 42:1372–1380. [Abstract] [Google Scholar]
- Zhuo J, Alcorn D, Harris PJ, Mendelsohn FA (1993) Localization and properties of angiotensin II receptors in rat kidney. Kidney Int Suppl 42:S40–S46. [Abstract] [Google Scholar]
- Zhuo J, Alcorn D, McCausland J, Casley D, Mendelsohn FA (1994) In vivo occupancy of angiotensin II subtype 1 receptors in rat renal medullary interstitial cells. Hypertension 23:838–843. [Abstract] [Google Scholar]
- Zhuo J, Dean R, MacGregor D, Alcorn D, Mendelsohn FA (1996) Presence of angiotensin II AT2 receptor binding sites in the adventitia of human kidney vasculature. Clin Exp Pharmacol Physiol 23 (Suppl 3):S147–S154. [Abstract] [Google Scholar]
- Zhuo JL, Imig JD, Hammond TG, Orengo S, Benes E, Navar LG (2002) Ang II accumulation in rat renal endosomes during Ang II-induced hypertension: role of AT(1) receptor. Hypertension 39:116–121. [Abstract] [Google Scholar]
- Zhuo JL, Li XC, Garvin JL, Navar LG, Carretero OA (2006) Intracellular ANG II induces cytosolic Ca2+ mobilization by stimulating intracellular AT1 receptors in proximal tubule cells. Am J Physiol Renal Physiol 290:F1382–F1390. [Europe PMC free article] [Abstract] [Google Scholar]
- Ziegler CG, Allon SJ, Nyquist SK, Mbano IM, Miao VN, Tzouanas CN, Cao Y, Yousif AS, Bals J, Hauser BM (2020) SARS-CoV-2 receptor ACE2 is an interferon-stimulated gene in human airway epithelial cells and is detected in specific cell subsets across tissues. Cell 181:1016–1035.e19. [Europe PMC free article] [Abstract] [Google Scholar]
- Zimmerman MA, Baban B, Tipton AJ, O’Connor PM, Sullivan JC (2015) Chronic ANG II infusion induces sex-specific increases in renal T cells in Sprague-Dawley rats. Am J Physiol Renal Physiol 308:F706–F712. [Europe PMC free article] [Abstract] [Google Scholar]
Articles from Pharmacological Reviews are provided here courtesy of American Society for Pharmacology and Experimental Therapeutics
Full text links
Read article at publisher's site: https://doi.org/10.1124/pharmrev.120.000236
Read article for free, from open access legal sources, via Unpaywall:
https://pharmrev.aspetjournals.org/content/pharmrev/74/3/462.full.pdf
Citations & impact
Impact metrics
Citations of article over time
Alternative metrics

Discover the attention surrounding your research
https://www.altmetric.com/details/129781186
Smart citations by scite.ai
Explore citation contexts and check if this article has been
supported or disputed.
https://scite.ai/reports/10.1124/pharmrev.120.000236
Article citations
Functional consequences of spatial, temporal and ligand bias of G protein-coupled receptors.
Nat Rev Nephrol, 20(11):722-741, 22 Jul 2024
Cited by: 0 articles | PMID: 39039165
Review
Angiotensin-(1-12): Does It Exist? A Critical Evaluation in Humans, Rats, and Mice.
Hypertension, 81(8):1776-1784, 08 May 2024
Cited by: 0 articles | PMID: 38716648 | PMCID: PMC11251504
Angiotensin II Directly Increases Endothelial Calcium and Nitric Oxide in Kidney and Brain Microvessels In Vivo With Reduced Efficacy in Hypertension.
J Am Heart Assoc, 13(10):e033998, 10 May 2024
Cited by: 1 article | PMID: 38726925 | PMCID: PMC11179802
Targeting Angiotensinogen With N-Acetylgalactosamine-Conjugated Small Interfering RNA to Reduce Blood Pressure.
Arterioscler Thromb Vasc Biol, 43(12):2256-2264, 19 Oct 2023
Cited by: 2 articles | PMID: 37855126 | PMCID: PMC10659251
Review Free full text in Europe PMC
New Approaches Targeting the Renin-Angiotensin System: Inhibition of Brain Aminopeptidase A, ACE2 Ubiquitination, and Angiotensinogen.
Can J Cardiol, 39(12):1900-1912, 20 Jun 2023
Cited by: 3 articles | PMID: 37348757 | PMCID: PMC10730775
Review Free full text in Europe PMC
Go to all (12) article citations
Data
Data behind the article
This data has been text mined from the article, or deposited into data resources.
Clinical Trials
- (1 citation) ClinicalTrials.gov - NCT04335136
Similar Articles
To arrive at the top five similar articles we use a word-weighted algorithm to compare words from the Title and Abstract of each citation.
Megalin-Mediated Endocytosis in the Kidney Proximal Tubule: Relevance to Regulation of the Renal Renin-Angiotensin System.
Nephron, 147(3-4):244-249, 12 Sep 2022
Cited by: 1 article | PMID: 36096093
Review
Recent Updates on the Proximal Tubule Renin-Angiotensin System in Angiotensin II-Dependent Hypertension.
Curr Hypertens Rep, 18(8):63, 01 Aug 2016
Cited by: 19 articles | PMID: 27372447 | PMCID: PMC5578446
Review Free full text in Europe PMC
The evolving complexity of the collecting duct renin-angiotensin system in hypertension.
Nat Rev Nephrol, 17(7):481-492, 06 Apr 2021
Cited by: 20 articles | PMID: 33824491 | PMCID: PMC8443079
Review Free full text in Europe PMC
The intrarenal renin-angiotensin system: from physiology to the pathobiology of hypertension and kidney disease.
Pharmacol Rev, 59(3):251-287, 01 Sep 2007
Cited by: 738 articles | PMID: 17878513
Review
Funding
Funders who supported this work.
NIAID NIH HHS (1)
Grant ID: R21 AI166940
NIDDK NIH HHS (3)
Grant ID: R01 DK067299
Grant ID: R01 DK102429
Grant ID: R01 DK123144