Abstract
Free full text

The translating bacterial ribosome at 1.55
Å resolution generated by cryo-EM imaging services
Abstract
Our understanding of protein synthesis has been conceptualised around the structure and function of the bacterial ribosome. This complex macromolecular machine is the target of important antimicrobial drugs, an integral line of defence against infectious diseases. Here, we describe how open access to cryo-electron microscopy facilities combined with bespoke user support enabled structural determination of the translating ribosome from Escherichia coli at 1.55Å resolution. The obtained structures allow for direct determination of the rRNA sequence to identify ribosome polymorphism sites in the E. coli strain used in this study and enable interpretation of the ribosomal active and peripheral sites at unprecedented resolution. This includes scarcely populated chimeric hybrid states of the ribosome engaged in several tRNA translocation steps resolved at ~2
Å resolution. The current map not only improves our understanding of protein synthesis but also allows for more precise structure-based drug design of antibiotics to tackle rising bacterial resistance.
Introduction
Cryo-electron microscopy (cryo-EM) allows for detailed structural determination of biomolecules, and it is currently the method of choice for obtaining high-resolution reconstructions of large and conformationally heterogenous macromolecular complexes vitrified in functional buffers after purification or directly within their native cellular context1–3. Although a remarkably powerful tool for structural biologists, cryo-EM experiments still rely on access to both high-end instrumentation and advanced expertise in sample preparation, data acquisition and image processing, thus posing major challenges for a growing number of life scientists that approach this method to tackle their biological questions for the first time. To address the ever-increasing demand for access to cryo-EM infrastructure and expertise, several local and national facilities have been established to provide access to cutting-edge transmission electron microscopes and project support by expert staff. To this end, the European Molecular Biology Laboratory (EMBL) has recently established the EMBL Imaging Centre, a new service unit whose main mission is to rapidly make the most advanced microscopy technologies available as a synergistic service portfolio to the international user community while providing comprehensive support from sample preparation to image acquisition and data analysis.
To demonstrate the capabilities of our cutting-edge instrumentation and experienced team to support external user projects requiring cryo-EM single particle analysis workflows, we vitrified, imaged, and analysed purified E. coli ribosomes stalled during translation. The bacterial 70S ribosome is an ideal candidate due to its fundamental importance in protein synthesis4–7 as well as being an active antibiotic target for antimicrobial research8. Furthermore, capturing intrinsic conformational states of the ribosomes, that usually represent challenges for structural biology approaches, is imperative to gain an atomic level understanding of the function of this intricate machine.
The ribosome has been a suitable target for single particle cryo-EM due to its large size, globular shape, and high RNA content, which generates relatively high contrast in the otherwise noisy cryo-EM images. Despite this, high-resolution structures (<2Å resolution) reported recently were limited to protein complexes with high symmetry such as apoferritin9–12, aldolase12 or β-galactosidase13. In contrast, the most detailed reconstructions of ribosomal complexes were so far resolved to 2.0
Å and 2.2
Å resolution, for the bacterial and the mammalian ribosomes14,15, respectively. This was mostly due to the lack of intrinsic symmetry of the ribosomes and their conformational and compositional heterogeneity, deriving from inter-subunit movements, flexible expansion rRNA segments, and the intermittent interactions with a plethora of additional translational factors involved in the different steps of translation.
Here, we describe how open access to state-of-the-art transmission electron microscopes combined with bespoke user support enabled structural determination of the bacterial translating ribosome at 1.55Å resolution. The structure, combined with previous insights, resolves many important features of the ribosome at atomic detail including chemical modifications, magnesium and potassium ions, as well as water molecules. The current resolution also allows for direct identification of multiple rRNA variations including a base pair swap in one of the rRNA helices that is distinct between different E. coli strains. The cryo-EM structure contained highly resolved features in the periphery of the ribosome including the ribosomal protein bL9, which provides insights on its possible function. Finally, the current dataset allowed us to resolve several conformational states of the ribosome with different tRNAs from a relatively small number of particles (~20,000 particles) at ~2
Å resolution.
Results
Isolation of translating ribosomes for cryo-EM structure determination
To isolate bacterial ribosomes actively engaged in translation, we employed a cell free in vitro translation PURE system16,17 of a mRNA encoding a FLAG-tagged nascent chain used for purification. The reaction mixture was incubated at 37οC to allow the ribosome to engage mRNA and start several elongation cycles, and after 30
min was placed on ice to pause translation. The translating ribosomes were isolated via a FLAG-tag affinity purification step followed by an ultracentrifugation step to pellet ribosomes from the preparation. Purified ribosomes were then applied to cryo-EM grids for imaging. We rationalised that the sample would contain translating ribosomes frozen at different functional states that can be further purified in silico by a combination of 3D image classification and focused 3D refinement approaches.
Cryo-EM sample screening at the EMBL Imaging Centre
To produce and identify a cryo-EM grid suitable for high-end data collection on a Titan Krios microscope, a total of 19 grids were vitrified over three freezing sessions and screened in two sessions using a Glacios (ThermoScientific) transmission electron microscope. At first, the screening was aimed at optimising sample spreading on the grid and ice thickness. Here, two different vitrification devices (ThermoScientific Vitrobot Mark IV and Leica EM GP2) and multiple different grid types (holey carbon grids with and without an additional layer of continuous carbon) were employed. Grid quality assessment was based on the low magnification grid overview which was acquired for each grid (commonly termed “grid map” or “grid atlas”) and high magnification screening images from those grids which showed promising ice thickness and had sufficient imageable areas for potential high-resolution data collection (Fig. 1 and Supplementary Fig. 1a, b).
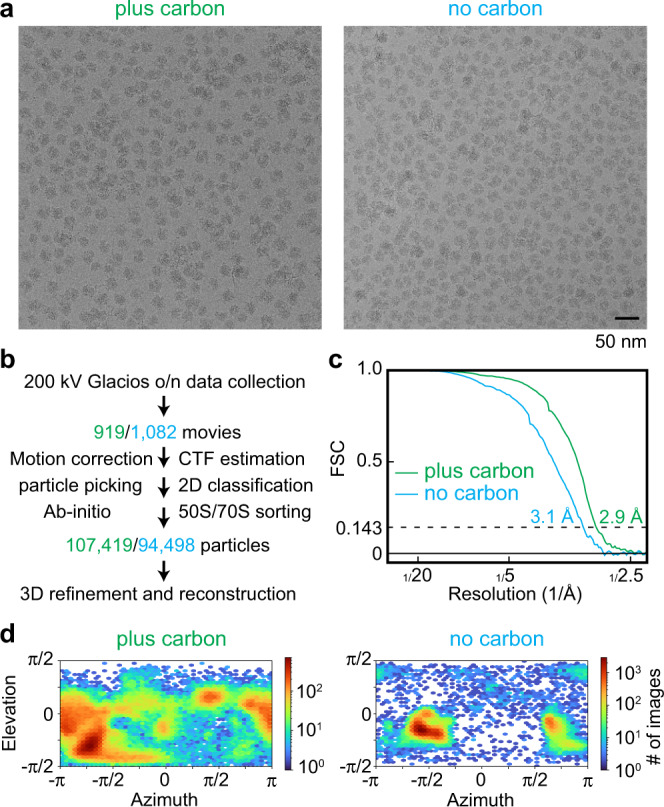
a Cryo-EM micrographs of the ribosome particles applied to holey carbon grids with (plus carbon, green) and without (no carbon, blue) an additional thin layer of continuous carbon (2nm). One micrograph out of 919 (plus carbon) or 1082 (no carbon) is shown. b Sample screening processing scheme using cryo-EM data collected overnight (o/n) on a ThermoScientific Glacios electron microscope. Colours as in “a”. c Fourier Shell Correlation (FSC) plots for the data collected on holey grids with and without an additional layer of continuous carbon. d Orientation distribution of the particles from data collected on plus and no carbon grids.
During the second stage of screening, the selected grids were assessed for ribosome particle density and distribution. A small overnight data set (~1000 movies) was then collected from the most promising grid at the end of each Glacios screening session. The two selected grids were a Quantifoil R2/1 grid with an additional 2nm layer of continuous carbon vitrified with the Vitrobot (“plus carbon” grid) and a Quantifoil R2/1 grid without the additional layer of continuous carbon vitrified with the EM GP2 using back side blotting to increase particle density in the foil holes (“no carbon” grid) (Fig. 1a and Supplementary Fig. 1b, c). Each dataset was analysed by employing the same processing workflow yielding 3D reconstructions of the 70
S ribosome at ~3
Å resolution from approximately 100,000 particles (Fig. 1b, c and Supplementary Fig. 1d). Despite the similar nominal resolutions obtained, the ‘plus carbon’ dataset had a substantially better particle orientation distribution compared to the “no carbon” dataset which suffered from severe preferred orientation (Fig. 1d). Therefore, we selected the ‘plus carbon’ grid for further high-end data collection on a Titan Krios G4 (ThermoScientific) equipped with a cold-FEG electron source, Selectris X energy filter and Falcon4 direct electron detector; over a 3-day session, a total of ~20,000 movies were collected.
High-resolution structure of P-site bound translating ribosome
We chose to process particles using a combination of both cryoSPARC18 and RELION19 software. Particle picking was done semi-automatically with crYOLO20 using the pre-trained general model, then followed by standard 2D classification and ab initio model generation which suggested the sample contained both translating ribosomes and free 50S subunit particles. Heterogeneous refinements in cryoSPARC were then employed to remove particles that belong to the free large ribosomal subunit and resulted in ~750,000 70
S ribosome particles. Further 3D classification resolved a class containing ~650,000 particles of a translating ribosome containing P-site tRNA and a weaker density for the E-site tRNA with the small 30
S subunit in the unrotated state (Supplementary Fig. 2–4). This is a widely observed state for translating ribosomes isolated using in vitro translation systems21–23. In this map, the P-site tRNA is tethered to a nascent chain (NC) density with weak density traceable to the backbone of the alpha carbons (Supplementary Fig. 5). However, clear densities of side chains were not observed in the polypeptide exit tunnel due to averaging of several states during translation and thus we did not fit an atomic model in this area.
A 3D variability analysis focused on the tRNA sites identified two subclasses with P- and E-site (~500,000 particles) and A-, P- and E-site (~150,000 particles) tRNA densities, respectively. A combination of 3D refinement schemes, contrast transfer function (CTF) refinement schemes as well as particle polishing19 were then used to obtain a final reconstruction to 1.55Å overall resolution of the larger class containing EM-densities corresponding to P- and E-site tRNAs (Supplementary Fig. 2 and 4, Supplementary Table 1). The local resolution of the small subunit was slightly lower (~1.9
Å) and was further improved to ~1.7
Å after performing focused 3D refinements and signal subtraction of the large subunit (Supplementary Fig. 2).
In the obtained structure, we can visualise rRNA modifications and protein side-chain conformers (Fig. 2) similar to those observed in a previously reported structure of the bacterial ribosome15 bound to mRNA and tRNA at 2.0Å. At the current resolution, we can observe separate EM-densities of water molecules coordinating magnesium ions bound to the RNA backbone (Fig. 2b). In addition, we modelled EM-densities for potassium ions (Fig. 2b), based on their identified positions in a recent X-ray structure24. Although the state of the ribosome is paused at different states during translation, we visualised the mRNA with defined anticodon-codon interactions being visible (Supplementary Fig. 6). It is thus likely that the ribosome is paused at preferred sites due to visible densities of purine and pyrimidine rings. However, a reliable assignment of the pausing site could not be assigned and is not further discussed here.
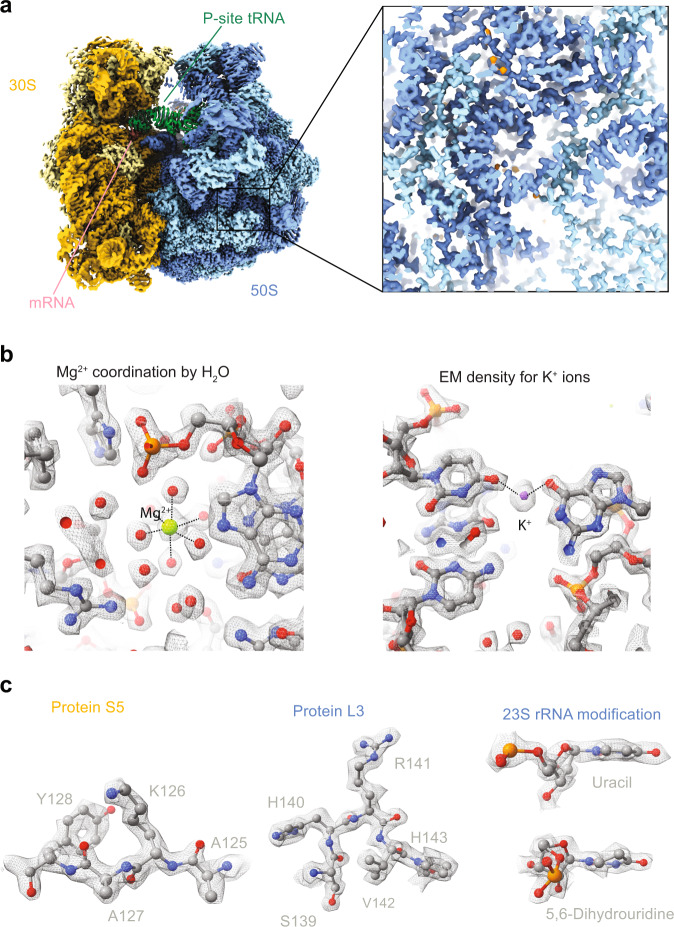


a Cryo-EM map of the bacterial translating ribosome at 1.55Å coloured in dark yellow (16
S rRNA), light yellow (ribosomal proteins in the small subunit), light blue (large subunit ribosomal proteins), dark blue (23
S and 5
S rRNA). b, c Closeup densities of protein side chain conformation, water molecules, magnesium, and rRNA modifications are shown as sticks with overlaid EM-densities shown as mesh. Density thresholds: 0.9 (Mg2+, b) and (K+, b), 0.5 (Protein S5, c), 0.65 (Protein L3, c) and 0.8 (23
S rRNA modification).
A C-G base-pair swap in the 23
S rRNA observed in the high-resolution map
Interestingly, we observed a swap between the E. coli MRE600 strain sequence of the 23S rRNA docked into our cryo-EM map. The initially identified swap was a G-C base-pair swap in the position G2210 and C2216 (MRE600 numbering) in helix 79 of the 23
S rRNA. After closer inspection, we realised that the PURE in vitro translation system kit was developed based on the E. coli B-strain instead25,26, of which the sequence fits well into the EM-density of our maps. Through sequence alignments of the seven different 23
S rRNA operons of the E. coli B-strain and comparing their fit to our EM density we could identify loci C2566_01480 and C2566_04790 as the predominant ones in our 70
S ribosome sample. There are two additional mutations within the same rRNA helix which fit to our density (Fig. 3a–c). All other differences between the MRE600 and B-strain rRNA sequences (mutations and insertions), are also reflected by our EM map. Sequence polymorphisms within the 23
S and 16
S rRNA is a common property of bacterial strains and environmental isolates of microbes which could be used as a possible indicator of strain virulence27,28. The C-G swap identified here is an intriguing example of co-evolution where two separate mutations in the 23
S rRNA sequence were attained to maintain the base-pairing and the integrity in the 23
S rRNA helix.
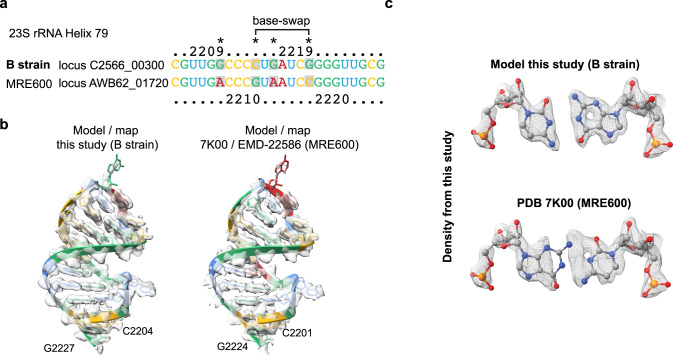

a Partial sequence alignment of H79 of the 23S rRNA between the E. coli B-strain and MRE600. Base-swap positioning is indicated. Other observed mutations are indicated by an asterisk. b Snapshot of H79 shown as cartoon illustration with base colouring as in “a”. Overlaid cryo-EM density is shown as transparent isosurface. c Close-up of the base pair swap using B-strain sequence and MRE600 sequence (PDB 7K00) with overlaid EM density shown as mesh.
Density corresponding to the ribosome protein bL9
In the current map, the spread of local resolution at the periphery is around 2–3Å (Supplementary Fig. 7a), which underscores the stability of our sample preparation and data processing schemes. We observe a well-resolved density at side-chain resolution of the ribosome proteins bL9 and uS6 (Fig. 4a–c, and Supplementary Fig. 7b, c). bL9 is located at a peripheral position of the ribosome forming a strut-like shape below the L1 stalk and bridging the large and the small subunits of the ribosome. The C-terminal domain of bL9 is highly mobile and was observed in an open conformation in previous X-ray structures29 and later observed in the closed conformation in high-resolution cryo-EM structures30 contacting the small ribosomal subunit. In the current structure, bL9 is observed in its entirety with the C-terminal domain establishing contacts with uS6 where it locks the L1 stalk in the closed conformation contacting the E-site tRNA (Fig. 4a). The closed conformation of this protein is stabilised via a salt bridge between Arg24 (uS6) and Glu87 (bL9) (Fig. 4c). Notably, the strut-like shape of bL9 is rich in negatively charged residues through its extended structure (Supplementary Fig. 7d), which would otherwise repel away from the ribosome. Furthermore, a mutant in the C-terminal domain (S93F) affects forward slippage on mRNA, suggesting a role for L9 in fidelity of translation31. We speculate that this unusual feature of a ribosomal protein carries an important function during protein synthesis that could also contribute to its detachment and flexibility in previous X-ray and cryo-EM structures that were done under variable salt conditions.
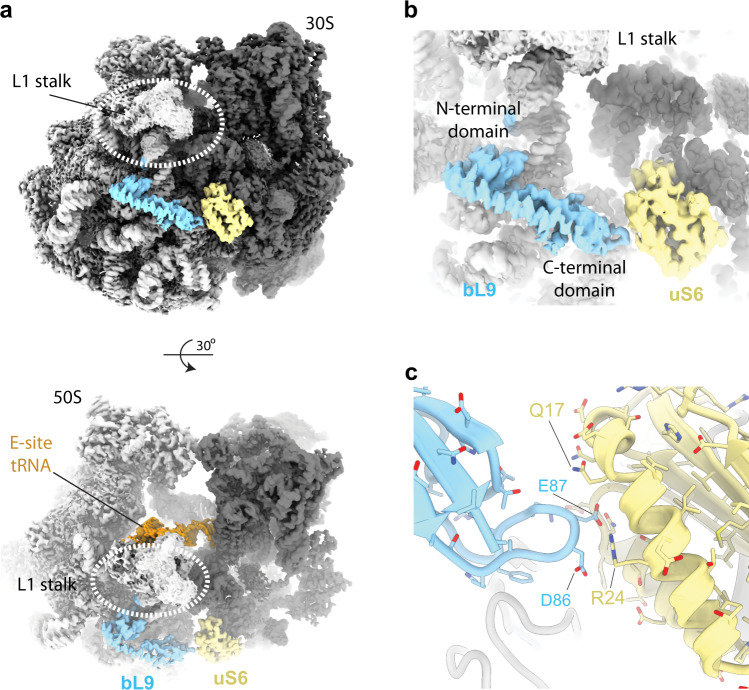

a bL9 (cyan) and uS6 (yellow) highlighted in the context of the cryo-EM map of the 70S ribosome. Large subunit is in light grey, small subunit in dark grey. L1 stalk of the ribosome is indicated. b, c Closeups of bL9 density with surrounding interactions with the small subunit. Ribosomal protein uS6 is indicated. Overlaid EM density is shown as a transparent surface in (b). Colouring as in (a).
The ribosomal protein bL9 plays an important role in influencing reading frame maintenance and restraining forward slippage on mRNA. This restraint can be circumvented by recoding signals that cause programmed frameshifting that are availed of by many viruses and mobile elements31–33. Recently, L9 interactions across neighbouring ribosomes translating the same mRNA were also shown to play a role in polysome formation34 and ribosome quality control pathways bridging interaction between two colliding ribosomes35,36. In addition, L9 interacts with elongation factor P (EF-P)37 as well as the ribosome biogenesis GTPases EngA38, extending the current known function of this protein. High-resolution of bL9 is thus highly pertinent to determining the outstanding functional aspects of this enigmatic dual-mode protein.
Multiple functional states of the translating bacterial ribosome
We also investigated the presence of additional functional states of the translating ribosome in the dataset. We employed 3D classification to identify a class of ribosomes in the rotated state which was further sub-classified using the 3D variability approach implemented in cryoSPARC by focusing on the tRNA binding sites on the ribosome (Fig. 5, Supplementary Fig. 2 and 3). This classification scheme resolved three tRNA translocation intermediates of the translating ribosomes. Besides the above described classical unrotated state with bound A-, P-, and E-site tRNAs, we also resolved two additional states containing chimeric state rotated ribosomes. The first class is of A/P- and P/E- tRNA hybrid state ribosomes and the second class with A- and P/E-site tRNAs (Fig. 5). The chimeric state was observed in the absence of the tRNA-bound elongation factor EF-Tu and was described in more detail in recent cryo-EM structures39–41. Interestingly, each of the two intermediates represent only ~2.7 % (~20, 000 particles) of the total dataset and yet could be refined to ~2.1Å indicating the possibility to sort for rare intermediates that can be resolved to high resolution from a relatively small number of particles.
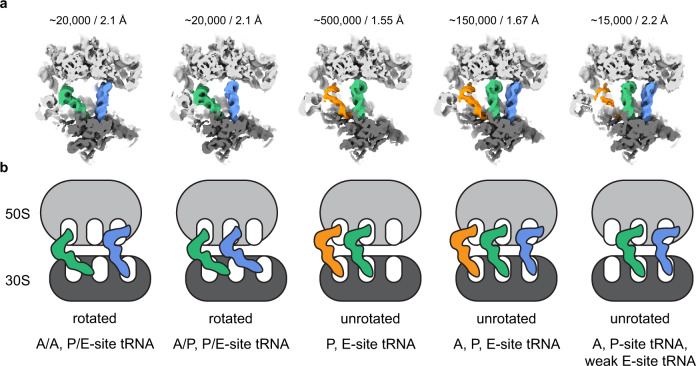
a Cryo-EM densities of different ribosome translating states from 3D variability analysis low-pass filtered at 5Å. Large ribosomal subunit coloured in light grey, small subunit in dark grey, A-site tRNA in blue, P-site in green, E-site in orange. Number of particles and resolution of the 3D reconstruction for each class are indicated above. b Schematic representation of all identified tRNA states in the data set. Colouring as in “a”. The respective tRNA state is indicated below.
Discussion
In summary, we present multiple structures of the E. coli translating ribosome, with the most populated state resolved at a global resolution of 1.55Å. To our knowledge, this is currently the highest resolution reported for the ribosome or any non-symmetrical complex by single particle cryo-EM. Determining structures of the ribosome has continuously progressed over the last 25 years since the initial X-ray structures emerged29,42–45. More recently, cryo-EM has provided insight into a plethora of functional states describing how ribosomes function, some of which were de-convoluted from the same reaction at high resolution. With current developments in cryo-EM image processing, direct pull-downs of active complexes are becoming the go-to approach requirement for resolving short-lived intermediates.
We suggest that a combination of improvements in sample preparation, microscopy hardware, data collection, and processing schemes with respect to previous studies allowed for this substantial advancement of the achievable resolution for an asymmetric and compositionally heterogeneous complex like the ribosome. The highly resolved rRNA in the presented structure allowed for sequence identification of portions of the rRNA directly from the map, where we detected a C-G base pair swap in H79 of the large subunit rRNA. This swap is due to sequence variation in the E. coli B-strain, compared to the MRE600 strain. Interestingly, despite the mutations being in two distant positions, the base pair swap does not alter the structural integrity of the helix. Such possible identification of base pair swapping in the rRNA could be used to identify bacterial strains by directly performing sequence analysis on their rRNA using cryo-EM performed on translating ribosomes purified from complex bacterial populations. Finally, the current dataset demonstrates how the latest generation of microscopy hardware and software allowed for the 3D classification and refinement of multiple conformational states of asymmetric complexes from a relatively small number of particles (~20,000) to around ~2Å. The results presented here show the importance of imaging facilities that provide open access to state-of-the-art instrumentation and tailored support to enable advanced structural studies pursued by researchers interested in cryo-EM as a new method.
Methods
Sample preparation
A synthetic DNA sequence (gBlock, IDT) with a T7 promoter, a leader region, and a Shine Dalgarno sequence upstream of sequence encoding an N-terminal 3x FLAG-tag followed by a 96 aa linker and sequence (373 nt) from bacteriophage MGS13 was used as template for PCR using primers gBlock S and gBlock AS (see Supplementary Table 2). PCR amplicons were verified by agarose gel electrophoresis and purified using the Monarch PCR and DNA Cleanup Kit (New England Biolabs). In vitro transcription was performed using the T7 RiboMAX Express Large Scale RNA Production System (Promega), followed by Lithium Chloride precipitation to purify the synthesized RNA.
In vitro translation was carried out using the deltaRF123 Kit (New England Biolabs). 200ng of mRNA was added per µL of reaction, with a total reaction volume of 1.5
mL. Reactions were incubated at 37
°C for 30
min. Translation reactions were chilled on ice for 10
min to halt translation, and HEPES KOH pH 7.4 was added to a final concentration of 50
mM. Chilled translation reactions were directly incubated with anti-FLAG M2 beads (Sigma) for 2
h at 4
°C with gentle mixing.
The beads were then washed with 50mM HEPES, pH 7.4, 100
mM KOAc, 25
mM Mg(OAc)2, 0.1% Triton X-100, 1
mM DTT; 50
mM HEPES, pH 7.4, 250
mM KOAc, 25
mM Mg(OAc)2, 0.5% Triton X-100, 1
mM DTT and RNC buffer (50
mM HEPES, pH 7.4, 100
mM KOAc, 25
mM Mg(OAc)2, 1
mM DTT). RNCs were eluted after 3 sequential 10
min incubations at room temperature in the RNC buffer that contained 0.2
mg/mL 3x FLAG peptide (Sigma). The elutions were combined and centrifuged at 186,000
×
g at 4
°C for 1.5
h in a (TLA-120.1) rotor (Beckman Coulter). Supernatant was discarded and the pellet was resuspended in the RNC buffer at a concentration of ~200
nM. At each step of translation reactions and purification, aliquots were taken to perform SDS-PAGE on 4–12% Bolt Polyacrylamide gels (Invitrogen) and Western blots. Gene products of interest were probed using a commercial anti-FLAG antibody (Sigma Cat. No. F1804).
Cryo-EM sample preparation
Cryo-EM grids have been prepared with a Vitrobot Mark IV (plus carbon sample, ThermoScientific) or an EM GP2 (no carbon sample, Leica) plunger. Environmental chambers were set to 6°C and 100% humidity. Grids were rendered hydrophilic by plasma cleaning for 30
s in a 90/10% Ar/O mixture with a Fischione 1070 plasma cleaner right before plunge freezing. 2.5
µL of the undiluted ribosome sample were applied to the foil side of the grid. The plus carbon sample (Quantifoil Cu300 R2/1
+
2
nm
C, as commercially available) was blotted for 2
s with blot force +3 after a 30
s wait time after sample application and immediately plunge frozen in liquid ethane. The no carbon sample (Quantifoil Cu200 R2/1) was blotted for 1.5
s from the back side right after sample application and immediately plunge frozen in liquid ethane. In all cases Whatman 597 blotting paper was used.
Cryo-EM data collection
Grid screening and screening data acquisition was performed on a Glacios TEM operated at 200kV equipped with a Selectris X energy filter and Falcon 4 direct electron detector (ThermoScientific). Data was acquired at a nominal magnification of 100 kx resulting in a calibrated pixel size of 1.154
Å on the camera. A 50
µm C2 and a 100
µm objective aperture were inserted and the width of the energy filter slit was set to 10
eV. The TEM was operated in nanoprobe mode at spot size 6. Movies were acquired over 8.3
s exposure time with a total accumulated dose of ~40 e-/Å2 (~6.4 e-/px/s over an empty area on the camera level) in counting mode and saved in the EER file format. For the plus carbon data set, 919 movies were acquired in one overnight data collection (1 exposure per hole/stage movement) and for the no carbon data set 1,082 movies were acquired in the same way.
After evaluation of the two screening data sets (see below), the plus carbon grid was selected for high-resolution data acquisition on a Titan Krios TEM operated at 300kV equipped with a C-FEG, Selectris X energy filter and Falcon 4 direct electron detector (ThermoScientific). A total of 19,449 movies were collected over three days at a nominal magnification of 165 kx resulting in a calibrated pixel size of 0.731
Å on the camera. A 50
µm C2 and a 100
µm objective aperture were inserted and the slit width of the energy filter was set to 10
eV. The TEM was operated in nanoprobe mode at spot size 5 and a beam diameter of ~460
nm. Movies were acquired over a 5.2
s exposure time with a total accumulated dose of ~40 e-/Å2 (~4.1 e-/px/s over an empty area on the camera level) in counting mode and saved in the EER file format (1293 fractions per movie). The target defocus was set to −0.5 to −1.5
µm with 0.1
µm steps between holes. In total, 16 movies were acquired per hole using beam image shift (11 in the outer ring, 5 in the inner ring) before moving to the next hole by stage shift. All TEM screening and data acquisition was performed using SerialEM46.
Data processing and model building
Cryo-EM data processing workflows for the Glacios screening data sets and the Krios high-resolution data set are shown in Fig. 1 and Supplementary Fig. 1, 2 and 3, respectively. Motion correction of EER movies was performed with the CPU implementation of MotionCor2 from Relion447. Initial CTF estimation was performed using CTFFIND448. Gautomatch (https://www2.mrc-lmb.cam.ac.uk/download/gautomatch-056/) was used for particle picking of the Glacios screening data, crYOLO20 with the pre-trained general model was used for picking of the high-resolution Krios data set. Particle extraction, 3D classification with alignment as well as Bayesian polishing were performed with Relion4. All other cryoEM data processing steps were performed in cryoSPARC18. Separation of 50S and 70
S particles was achieved via Heterogeneous Refinement, different tRNA states were identified using a combination of 3D classification and 3D Variability analysis. Maps were post-processed by local filtering after local resolution estimation as implemented in cryoSPARC. Conversion of cryoSPARC ‘.cs’ files to Relion ‘.star’ files was performed using UCSF pyem49 in combination with in-house written bash scripts50 (https://github.com/simonfromm/miscEM). All final Homogeneous Refinements were done with per-particle defocus and per-group CTF parameter optimization as well as Ewald Sphere Correction switched on. Exposures from all beam image shift positions of the acquisition pattern for the dataset collected on the Titan Krios were put into a separate group resulting in a total of 16 exposure/optics groups.
Model building was started with individually rigid-body fitting all chains from the published 2Å E. coli 70
S ribosome structure (PDB 7K00) in COOT51. After manual inspection of the rRNA of the large and small ribosomal subunits for mutations, the residue numbering of the E. coli B-strain was used. All chains were inspected and adjusted manually in COOT and subsequently refined with real-space refinement in PHENIX52 against the unsharpened map with secondary structure and Ramachandran restraints switched off (Supplementary Table 1). The C-terminal part of bL9 absent from PDB 7K00 was built de novo based on the AlphaFold2 model. Water molecules were added to the 30
S and 50
S part of the structure separately using “Find Waters” in COOT. Mg2+ and K+ positions were inferred from PDB 7K00 and 6QNR, respectively. All Mg2+ and K+ ion positions were checked and modified manually when necessary. The model geometry was validated using MolProbity53. The model vs. map FSC at FSC
=
0.5 coincides well with the one determined between the map half-sets at FSC
=
0.143 (Supplementary Fig. 4). All cryoEM density and model renderings were generated with ChimeraX54.
Reporting summary
Further information on research design is available in the Nature Portfolio Reporting Summary linked to this article.
Acknowledgements
We would like to thank members of the Mattei, Jomaa and Atkins labs for discussions. We are grateful to Dr. Jochen Zimmer for his comments on the manuscript. We also thank Maciej Gluc for discussions. This work was funded by the European Molecular Biology Laboratory to S.M., by the Department of Molecular Physiology and Biological Physics and the School of Medicine at the University of Virginia through a start-up grant to A.J., and by the Irish Research Council Advanced Laureate (IRCLA/2019/74) to J.F.A. This work benefited from access to EMBL Imaging Centre and has been supported by iNEXT-Discovery, project number 871037, funded by the Horizon 2020 program of the European Commission. We acknowledge the access and services provided by the Imaging Centre at the European Molecular Biology Laboratory (EMBL IC), generously supported by the Boehringer Ingelheim Foundation.
Author contributions
S.M., A.J. and J.F.A. conceived the project, K.O.C., P.R.B., G.L. purified the ribosomes. S.A.F. screened and collected cryo-EM data. S.A.F., A.J., and M.P. processed the cryo-EM data. S.A.F built the model with input from A.J. and S.M. S.M., A.J. and J.F.A. supervised the work. A.J., S.A.F., and S.M. wrote the manuscript. All authors contributed to the final version of the manuscript.
Peer review
Peer review information
Nature Communications thanks the anonymous reviewer(s) for their contribution to the peer review of this work.
Data availability
The maps and models generated in this study have been deposited in the EM and protein database under accession codes EMD-15793 (high-resolution structure), EMD-15794 (rotated, A/A an P/E tRNAs), EMD-15795 (rotated, A/P and P/E tRNAs), EMD-15796 (unrotated, A and P tRNAs), EMD-15797 (unrotated, A, P and E tRNAs) and PDB 8B0X (high-resolution structure). All micrographs and coordinates for the picked particles have been deposited in Electron Microscopy Public Image Archive under accession code EMPIAR-11377.
Footnotes
Publisher’s note Springer Nature remains neutral with regard to jurisdictional claims in published maps and institutional affiliations.
These authors contributed equally: Simon A. Fromm, Ahmad Jomaa.
Contributor Information
John F. Atkins, Email: [email protected].
Ahmad Jomaa, Email: ude.ainigriv@aamojdamha.
Simone Mattei, Email: [email protected].
Supplementary information
The online version contains supplementary material available at 10.1038/s41467-023-36742-3.
References
Articles from Nature Communications are provided here courtesy of Nature Publishing Group
Full text links
Read article at publisher's site: https://doi.org/10.1038/s41467-023-36742-3
Read article for free, from open access legal sources, via Unpaywall:
https://www.nature.com/articles/s41467-023-36742-3.pdf
Citations & impact
Impact metrics
Citations of article over time
Alternative metrics

Discover the attention surrounding your research
https://www.altmetric.com/details/142918663
Smart citations by scite.ai
Explore citation contexts and check if this article has been
supported or disputed.
https://scite.ai/reports/10.1038/s41467-023-36742-3
Article citations
Structure of Aquifex aeolicus lumazine synthase by cryo-electron microscopy to 1.42 Å resolution.
IUCrJ, 11(pt 5):723-729, 01 Sep 2024
Cited by: 0 articles | PMID: 38965901 | PMCID: PMC11364023
Rumicidins are a family of mammalian host-defense peptides plugging the 70S ribosome exit tunnel.
Nat Commun, 15(1):8925, 16 Oct 2024
Cited by: 0 articles | PMID: 39414793 | PMCID: PMC11484942
Chemical strategies for antisense antibiotics.
Chem Soc Rev, 22 Oct 2024
Cited by: 0 articles | PMID: 39436264 | PMCID: PMC11495246
Review Free full text in Europe PMC
Metastasis-Associated Lung Adenocarcinoma Transcript 1 (<i>MALAT1</i>) lncRNA Conformational Dynamics in Complex with RNA-Binding Protein with Serine-Rich Domain 1 (RNPS1) in the Pan-cancer Splicing and Gene Expression.
ACS Omega, 9(41):42212-42226, 03 Oct 2024
Cited by: 0 articles | PMID: 39431102 | PMCID: PMC11483381
CheckMyMetal (CMM): validating metal-binding sites in X-ray and cryo-EM data.
IUCrJ, 11(pt 5):871-877, 01 Sep 2024
Cited by: 0 articles | PMID: 39141478 | PMCID: PMC11364027
Go to all (13) article citations
Data
Data behind the article
This data has been text mined from the article, or deposited into data resources.
BioStudies: supplemental material and supporting data
Protein structures in PDBe (2)
-
(4 citations)
PDBe - 7K00View structure
-
(1 citation)
PDBe - 6QNRView structure
Electron Microscopy Data Bank (EMDB) at PDBe (5)
Similar Articles
To arrive at the top five similar articles we use a word-weighted algorithm to compare words from the Title and Abstract of each citation.
Structure of the E. coli ribosome-EF-Tu complex at <3 Å resolution by Cs-corrected cryo-EM.
Nature, 520(7548):567-570, 23 Feb 2015
Cited by: 224 articles | PMID: 25707802
Cryo-electron microscopy structure and translocation mechanism of the crenarchaeal ribosome.
Nucleic Acids Res, 51(17):8909-8924, 01 Sep 2023
Cited by: 2 articles | PMID: 37604686 | PMCID: PMC10516650
Structure of the bacterial ribosome at 2 Å resolution.
Elife, 9:e60482, 14 Sep 2020
Cited by: 135 articles | PMID: 32924932 | PMCID: PMC7550191
Focused classification and refinement in high-resolution cryo-EM structural analysis of ribosome complexes.
Curr Opin Struct Biol, 46:140-148, 29 Aug 2017
Cited by: 28 articles | PMID: 28850874
Review
Funding
Funders who supported this work.