Abstract
Free full text

GABA shunt pathway is stimulated in response to early defoliation-induced carbohydrate limitation in Mandarin fruits
Associated Data
Abstract
The regulation of sugar and organic acid metabolism during fruit development has a major effect on high-quality fruit production. The reduction of leaf area is a common feature in plant growth, induced by abiotic and biotic stresses and disturbing source/sink ratio, thus impacting fruit quality. Here, we induced carbohydrate limitation by partial leaf defoliation at the beginning of the second stage of mandarin development (before the citrate peak). Resulting changes were monitored in the short-term (48 h and 1 week) and long-term (7 weeks) after the defoliation. Short-term response to early defoliation implied metabolic settings to re-feed TCA for sustaining respiration rate. These features involved (i) vacuolar sucrose degradation (high acid invertase activity and mRNA expression level) and enhanced glycolytic flux (high ATP-phosphofructokinase activity), (ii) malic and citric acid utilization (increased phosphoenolpyruvate kinase and NADP-Isocitrate dehydrogenase) associated with vacuolar citric acid release (high mRNA expression of the transporter CsCit1) and (iii) stimulation of GABA shunt pathway (low GABA content and increased mRNA expression of succinate semialdehyde dehydrogenase). A steady-state proline level was found in ED fruits although an increase in P5CS mRNA expression level. These results contribute to a better knowledge of the molecular basis of the relationship between defoliation and sugar and organic acid metabolism in mandarin fruit.
1. Introduction
Citrus crops are one of the world's most widespread commercial crops. The Mediterranean region is one of the main producers, especially for mandarins [1]. Citrus fruits contain a large variety of primary metabolites including soluble sugars, organic, amino, and fatty acids, and various secondary metabolites like flavonoids and carotenoids that determine organoleptic quality and contribute to the nutritional traits and health benefits of citrus fruits. Citrus fruit ripening is divided into three stages. Stage I is characterized by a slow rate of fruit growth but high cell division and takes place over 2 months. Then, stage II corresponds to a fast growth period with a sharp increase in fruit size mainly due to cell expansion and water accumulation. This phase usually lasts between 4 and 6 months. Finally, in stage III, growth slows down and most of the transformations characteristic of fruit maturation and ripening take place, including color changes [2]. Among primary metabolites, carbohydrates account for 80% of the total soluble solids; sucrose, glucose and fructose are the major soluble sugars in citrus fruit [3,4]. During fruit development, soluble sugar content increases and sucrose, glucose and fructose accumulate in a ratio of 2:1:1 in many citrus cultivars. Citric acid is the major organic acid that accumulates in citrus fruits; it accounts for between 70% and 90% of total acids [5], with malic acid being second (between 10% and 15%). Citric acid accumulation begins during the second stage of citrus fruit development, reaches a peak and then gradually decreases as the fruit matures. Moreover, it has been reported that citric acid content is correlated with the total acidity of citrus fruits [6]. Since soluble sugars and organic acids, especially citric acid, are key components of fruit taste and quality, their amount and metabolism are a relevant trait for the citrus market.
The metabolism of organic acids is closely linked to sugar metabolism. Sucrose— the major photo-assimilate found in the citrus phloem—can be hydrolyzed by cytosolic invertase or stored in the acidic vacuoles and hydrolyzed by a vacuolar acid invertase [7]. Then, an irreversible reaction catalyzed by phosphofructokinase (PFK) is a major regulating step of the glycolysis pathway, which leads to the formation of phosphoenolpyruvate (PEP). The acidity development is initiated by malate and oxaloacetate (OAA) synthesis. Cytosolic phosphoenolpyruvate carboxylase (PEPC) catalyzes PEP carboxylation to produce OAA, which can be reduced to malic acid by cytosolic malate dehydrogenase (MDH), or utilized in the TCA cycle in mitochondria [8]. Citric acid accumulation in citrus fruits is determined by three mechanisms: (i) synthesis in mitochondria, (ii) storage in the vacuole, and (iii) catabolism in cytoplasm [9,10]. Citric acid is formed from the TCA cycle through the condensation of acetyl-CoA and OAA, catalyzed by citrate synthase. Citric acid content declines during the second half of citrus fruit ripening. Shimada et al. (2006) showed a relationship between decreased citrate content and CsCit1 activity, an H+/citrate vacuolar symporter. In the cytoplasm, citrate degradation can be targeted for amino acid biosynthesis such as glutamine or proline. It has been shown that glutamate can be utilized for glutamine production or catabolized through the γ-aminobutyric acid (GABA) shunt pathway [11]. Furthermore [12], showed that the highest expression of genes encoding three enzymes that make up the GABA shunt (glutamate decarboxylase, GABA aminotransferase, succinate semialdehyde dehydrogenase (SSADH)) was observed during the citrate decline stage.
During citrus fruit development, sugar and organic acid metabolism, and their accumulation, can be affected by environmental factors [6], genetic factors [3], growing practices including irrigation [13] and source/sink ratio [14], which could have an impact on fruit quality.
Source/sink ratio can be modified by sink limitations that depend on organ genetic features and developmental stage, while source limitations can be affected by environmental conditions [15]. For example, drought stress leads to a low source/sink ratio due to the reduced production of photo-assimilates, impacting fruit growth and quality [16]. Carbohydrate limitation has been reported to reduce fruit size and quality in muskmelon [17], grape berries [18] and tomatoes [19]. Source/sink ratio can also be modified by partial defoliation which has a significant effect on the metabolism of different species including grapevine [20], mango [21] and orange [22]. Partial defoliation leads to a source/sink imbalance, thereby inducing changes in soluble sugar and organic acid accumulation [14,23,24].
A previous study has demonstrated that (i) defoliation outcomes depend on the timing of leaf removal during citrus fruit growth stages, (ii) early defoliation significantly reduced fruit growth (fresh weight and fruit diameter) at the end of the stage III, amount and utilization of primary metabolites, including sucrose, organic and amino acids, (iii) the short-term defoliation response is characterized by sustained hexose content near to control values [14]. Hence, in this study, we aim to decipher the main pathways involved in this short-term defoliation response to sustain carbohydrate content. Early defoliation was applied in mandarin fruits at the beginning of stage II of fruit development, before the citrate peak. We integrated biochemical data (sugar and organic acid content and enzymes involved in their metabolism) and gene expression analysis that allowed us to determine the molecular mechanisms underlying changes in mandarin fruit composition induced by early defoliation.
2. Material and methods
2.1. Experimental sites, growth and sampling conditions
Data were recorded on fruit grown on 18-years-old mandarin trees (Citrus reticulata Blanco cv Willowleaf) of the Inrae-Cirad Citrus Biological Resource Centre of San Giuliano in Corsica (42°18′55”N, 9°29′29”E; 51 m above sea level). Poncirus trifoliata (L) Raf. was used as rootstock for all scions. Trees were grown under the same conditions as previously described by Ref. [14]. Water was supplied every day on the basis 100% replacement of actual evapotranspiration estimated from the Penman-Monteith equation [25]. Fertilizers were provided to meet standard conditions. Four trees were selected for this experiment and the treatments were randomly distributed in the plot. Only fully expanded flowers from May were selected to obtain fruit of similar age. The treatments were applied in July on the tree plants after fruit abscission in June.
Selected branches were composed of shoots less than 1 year-old. All branches had the same south-eastern orientation and similar light exposure. Girdling was applied on shoots with at least 30 leaves at 90 days of development after fruit set. Girdling consisted of removing a 10-mm-wide band of bark in the middle of the main stem of each selected branch to prevent any movement of assimilates between the fruiting branch and the rest of the tree. We checked that no phloem connection reformed after girdling. All girdled fruiting branches had only one fruit and 30 leaves at the beginning of the trial. For the control conditions, we removed fruit and leaves, if needed, to obtain 30 leaves per fruit which were chosen to avoid limitation of carbon supply. Early defoliation (ED) consisted of removing 24 leaves after 113 days of development, at the beginning of the second stage, which corresponding to reducing the leaf-to-fruit ratio by 80%. Moreover, no fruit drop was observed during the trial. Four samples per treatment (control and ED) were harvested 48 h, 1 week and up to 7 weeks after ED was applied. Immediately after harvest, fruits were peeled, and the flesh was immediately frozen in liquid nitrogen and stored at −80 °C until used.
2.2. Metabolite assays
Four independent biological replicates of fruit samples from each tree and each time point (n = 4) were harvested for metabolite assays.
Soluble sugar (glucose, fructose and sucrose) and organic acid (malic and citric acids) content were assayed according to Ref. [3]. Briefly, 100 mg of lyophilized fruit powder were homogenized in 5 mL of 80% ethanol (v/v). Samples were centrifuged at 5000×g at 4 °C for 10 min. Soluble sugar and organic acid content were then analyzed by HPLC [3,14].
Measurements of proline content were carried out according to Ref. [26]. Fruit pulp powder was homogenized in extraction buffer (10 mM HEPES-KOH, pH 7). Homogenates were centrifuged at 14,000×g at 4 °C for 5 min. Absorbance was read at 520 nm and proline content was determined using a standard curve.
For γ-aminobutyric acid (GABA) content, extraction and derivatization were performed according to Ref. [27] modified for citrus sample by Ref. [14]. The homogenates were then collected in a glass insert (100 μL) into an amber vial for further HPLC profiling.
2.3. Enzyme activities
2.3.1. Sample preparation
Aliquots of 10 to 20 mg FW were extracted by vigorous vortexing with extraction buffer [28]. The composition of the extraction buffer was 10% (v/v) glycerol, 0.25% (w/v) BSA, 0.1% (v/v) Triton X-100, 50 mM HEPES/KOH pH 7.5, 10 mM MgCl2, 1 mM EDTA, 1 mM EGTA, 1 mM benzamidine, 1 mM e-aminocaproic acid, 1 mM phenylmethylsulfonyl fluoride, 10 mM leupeptin, and 1 mM DTT. Phenylmethylsulfonyl fluoride was added just before extraction. The homogenate was centrifuged at 14,000×g for 5 min at 4 °C and the supernatant was used for enzyme analysis.
2.3.2. Phosphofructokinase (EC 2.7.1.11) assay
Phosphofructokinase (PFK) activity was determined using a method modified from [29]. Briefly, 2 μL of the extract was added to 500 mM Tricine-KOH buffer (pH 8.5) containing 25 Mm MgCl2, 0.25 mM NADH, 7.5 mM fructose-6-phosphate, 1 U mL−1 aldolase, 1 U mL−1 triose phosphate isomerase, 1 U mL−1 glycerol-3-phosphate dehydrogenase. The reaction was started by adding ATP to a final concentration of 0 (blank) or 0.25 mM (maximal activity). The reaction was stopped with 20 μL of 0.5 M HCl after incubation for 20 min and then 20 μL of NaOH 0.5 M were added to adjust the pH to 9. Glycerol-3-phosphate was measured as described in Ref. [30] in the presence of 1.8 units.mL−1 glycerol-3-phosphate oxidase, 0.7 unit.mL−1 glycerol-3-phosphate dehydrogenase, 1 mM NADH, 1.5 mM MgCl2, and 100 mM Tricine-KOH (pH 8.0). The absorbance was red at 340 nm and at 30 °C until rates were stabilized. Reaction rates were then used to calculate the amount of fructose-bisphosphate produced by the reaction, which enabled to calculate the activity.
2.3.3. NADP-dependent isocitrate dehydrogenase (EC 1.1.1.41) assay
NADP-dependent isocitrate dehydrogenase (NADP-IDH) activity was determined according to a modified protocol [30]. Briefly, 2 μL of the extract was added to 100 mM Tricine-KOH buffer (pH 8.0) containing 4 mM MgCl2 and 1 mM NADP+. The reaction was started by adding isocitrate to a final concentration of 0 (blank) or 2 mM (maximal activity). The reaction was stopped with 20 μL of 0.5 M NaOH after incubation for 20 min. The plates were centrifuged (2 min at 2000 rpm), sealed and heated at 95 °C for 5 min. After cooling, 10 μL of HCl 1 M and 0.2 M Tricine-KOH (pH 9.0) were added to adjust the pH to 9. NADPH was measured in the presence of 3 units.mL−1 G6PDH grade I, 100 mM Tricine-KOH (pH 9.0), 5 mM MgCl2, 4 mM EDTA, 0.1 mM PMS, 0.6 mM MTT, and 3 mM glucose-6-phosphate. The absorbance was read at 570 nm and at 30 °C until the rates were stabilized. Reaction rates were then used to calculate the amount of NADPH produced by the reaction, which enabled to calculate the activity.
2.3.4. Phosphoenolpyruvate carboxylase (EC 4.1.1.31) assay
Phosphoenolpyruvate carboxylase (PEPC) activity was determined using a modified procedure [28]. Two μL of the extract was added in 100 mM Tricine-KOH buffer (pH 8.0) containing 20 mM MgCl2, 1 U mL−1 malate dehydrogenase, 10 mM NaHCO3 and 0.1 mM NADH. Contaminating NAD+ was removed from the NADH stock solution before the assay by heating for 10 min at 95 °C. The reaction was started by adding phosphoenolpyruvate to a final concentration of 0 (blank) or 2 mM (maximal activity). The reaction was stopped with 20 mL of 0.5 M HCl. The plates were mixed and centrifuged (2 min at 2000 rpm). They were then sealed using adhesive aluminum foil and heated at 95 °C for 10 min. After cooling, 10 μL of HCl 1 M and 0.2 M Tricine-KOH (pH 9.0) were added to adjust the pH to 9.0. NAD was measured in the presence of 6 units.mL−1 alcohol dehydrogenase (ADH), 100 mM Tricine-KOH (pH 9.0), 4 mM EDTA, 0.1 mM PES, 0.6 mM MTT, and 500 mM ethanol. Reaction rates were then used to calculate the amount of oxaloacetic acid produced by the reaction, which enabled to calculate the activity.
2.3.5. Acid invertase (EC 3.2.1.26) assay
Acid invertase (Acid INV) activity was determined according to the modified method of [31]. The reaction mixture (200 μL) contained 50 mM sodium acetate (pH 4.6) and 125 mM sucrose. The reaction was started by adding 10 μL of extract and then stopped by adding 40 μL 300 mM Tris (pH 9.0) after 5 min. For the blank, the extract was added when the pH was adjusted to 9. The quantity of glucose formed during the reaction was determined from the measured increase in NADH formation at 340 nm at 25 °C for 1 h (Kit Sigma-Aldrich, Steinheim, Germany).
2.4. Gene expression
2.4.1. RNA extraction
Total RNA was isolated from frozen tissue using the RNeasy Plant Mini Kit (Qiagen, Dusseldorf, Germany) and treated with RNase-free DNase (Qiagen) through column purification according to the manufacturer's instructions. RNA quality was assessed by spectrophotometry and gel electrophoresis.
2.4.2. Quantitative PCR analysis
RNA was extracted from juice sac cells with three biological replicates. First-strand cDNA was synthesized from 500 ng L−1 of total RNA with the RevertAid First Strand cDNA Synthesis Kit (Thermo scientific, Waltman, USA). Primer3 software (ver. 0.4.0; http://frodo.wi.mit.edu/primer3/) was used for primer design. Quantitative PCR was performed on the StepOne-Plus™ (Applied Biosystems, Foster City, CA, USA), using SYBR® Green (Quanta Biosciences, Gaithersburg, USA). A total reaction volume of 15 μL was used. The reaction mix included 2 μL cDNA, 0.4 μL of reverse primer, 0.4 μL of forward primer, 10 μL PerfeCTa® SYBR® Green SuperMix ROX™, and 4.2 μL RNA-free water. A qPCR assay was performed using the following conditions: 95 °C for 5 min followed by 40 cycles of 95 °C for 15s and 60 °C for 40 s. The 2-ΔΔCT method24 was used to normalize and calibrate transcript values relative to the endogenous citrus actin gene, whose expression does not change across citrus fruit developmental stages. Primer sequences are described in Table 1.
Table 1
Primers used for mRNA expression by q-PCR.
Functions | Abbreviation | Forward and Reverse primers | |
---|---|---|---|
Metabolism | Invertase | INV | 5′-GCTCAAGGTGGGAGAACAAC |
3′-GTATGGACGGATGAATGCAG | |||
Succinate semialdehyde deshydrogenase | SSADH | 5′-ATGACGTTGAGTGGAGTGGGCA | |
3′-ATCTGGCGAGAGAAAGCAGGA | |||
Δ1-pyrroline-5-carboxylate synthetase | P5CS | 5′-ATGTGCGTGCTGCTATTGAC | |
3′-AGTCCAAATCGTGCTCCATC | |||
Transport | Citrate transporter | CsCit1 | 5′-GCTGCTCTGGCCTTTCTAAT |
3′-TGCCTGTTACGGAGACTTGA | |||
Control | Actin | ACT | 5′-CAGTGTTTGGATTGGAGGATCA |
3′-TCGCCCTTTGAGATCCACAT |
2.5. Statistical analyses
All data were analyzed using the R and RStudio statistical software (http://www.R-project.org, accessed on July 20, 2022) and Rstudio (Rstudio Inc., Boston, MA, USA, version April 1, 1717). All data were expressed as mean values (± standard error) and submitted to two-way ANOVA with an LSD test when a significant difference was detected at p < 0.05. A two-way hierarchical clustering classification was performed on normalized data (Ward's method) and presented as heat maps, using the gplot package and heatmap.2 function.
3. Results
3.1. Changes in sugar metabolism after early defoliation
Early defoliation induced an increase in sucrose content 48 h after defoliation, whereas a significant decrease of 20% and 9% was observed 1 and 7 weeks after defoliation, respectively, in comparison with control (Fig. 1A). Higher glucose and fructose content were observed in the ED fruits than the control fruits, 48 h after defoliation (Fig. 1B; 1C). Then, a decrease was found in glucose and fructose content 1 week after defoliation. While glucose content was greater in the ED fruits than the control fruits 7 weeks after defoliation, fructose content was still lower in ED fruits than control.

Concentrations of (A) sucrose, (B) glucose, and (C) fructose, changes in (D) phosphofructokinase (PFK), and (E) acid invertase (acid INV) activities, and (F) relative expression level of gene encoding acid INV, measured 48h, 1 week, and 7 weeks following defoliation in fruits under control (blue bars) and early defoliation (ED) (orange bars) treatments. For each parameter, the corresponding period in the fruit growth is also given in days after anthesis. Data are mean values (±SD) of four independent measurements (n = 4). Different lowercase letters indicate significant differences between treatment and along the development stages (P < 0.05). (For interpretation of the references to color in this figure legend, the reader is referred to the Web version of this article.)
PFK activity was 2.2-fold higher 48 h after defoliation in ED fruit compared to control (Fig. 1D). No significant change was observed 1 week and 7 weeks after defoliation. Acid INV activity rose sharply in fruits subjected to ED, 48 h (33%), 1 week (40%) and 7 weeks (37%) after defoliation (Fig. 1E) while the expression of mRNA encoding acid INV was significantly greater in the ED fruits 1 week and 7 weeks after defoliation in comparison with control (Fig. 1F).
3.2. Changes in organic acid metabolism after early defoliation
Fruits subjected to defoliation, as control, displayed a slight decline in malic acid content during fruit development (Fig. 2A). While citric acid content remained constant during fruit development in control fruits, ED fruits showed a decline before reaching the same value as control at the end of the study (Fig. 2B).
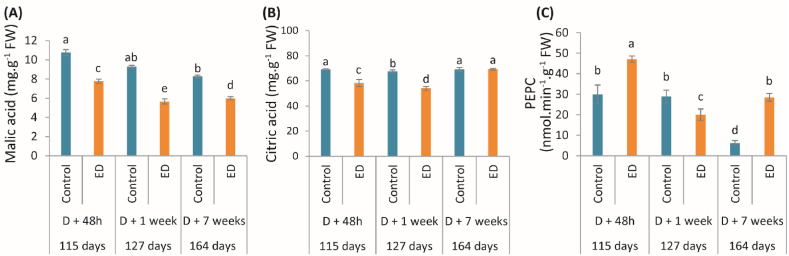
Concentrations of (A) malic acid, and (B) citric acid, and changes in (C) phosphoenolpyruvate kinase (PEPC) activity measured at 48h, 1 week, and 7 weeks following defoliation in fruits under control (blue bars) and early defoliation (ED) (orange bars) treatments. For each parameter, the corresponding period in the fruit growth is also given in days after anthesis. Data are mean values (±SD) of four independent measurements (n = 4). Different lowercase letters indicate significant differences between treatment and along the development stage (P < 0.05). (For interpretation of the references to color in this figure legend, the reader is referred to the Web version of this article.)
Early defoliation induced significant changes in both malic and citric acid levels in fruits (Fig. 2A; B). The malic acid content was lower in fruits subjected to early defoliation, in comparison to the controls; a sharp reduction was also observed 1 week after early defoliation (39%) (Fig. 2A). The ED fruits showed a decline of citric acid content 48 h (15%) and 1 week (19%) after defoliation, whereas no significant change was observed 7 weeks after defoliation.
PEPC activity decreased during fruit development in control and ED fruits, which showed an increase at the end of the experiment (Fig. 2C). Overall, early defoliation induced an increase in PEPC activity during fruit development. The highest PEPC activity was observed in ED fruit 48 h after defoliation. While a decline of PEPC activity was found between the control and ED fruits 1 week after defoliation, PEPC activity was 1.6-fold and 4.6-fold greater 48 h and 7 weeks after defoliation, respectively (Fig. 2C).
3.3. Vacuolar release and fate of citrate in the cytosol through GABA shunt pathways after early defoliation
The qPCR analysis showed a sharp increase in mRNA encoding CsCit1, in response to early defoliation (Fig. 3A). The relative expression level was 2.2-fold and 2.8-fold higher in the ED fruits, 48 h and 1 week after defoliation, respectively. A decline between the ED fruits and the control was detected after 7 weeks.
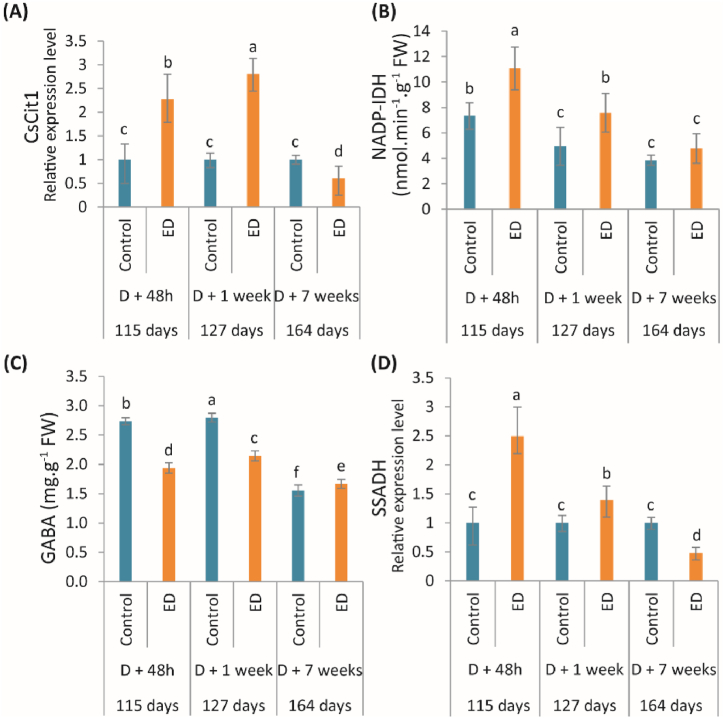
(A) Relative expression level of the gene encoding the vacuolar transporter CsCit1, changes in (B) NADP-IDH activity, and (C) GABA content, and (D) relative expression level of the gene encoding SSADH, measured at 48h, 1 week, and 7 weeks following defoliation in fruits under control (blue bars) and early defoliation (ED) (orange bars) treatments. For each parameter, the corresponding period in the fruit growth is also given in days after anthesis. Data are mean values (±SD) of four independent measurements (n = 4). Different lowercase letters indicate significant difference between treatment and along the development stages (P < 0.05). (For interpretation of the references to color in this figure legend, the reader is referred to the Web version of this article.)
NADP-IDH activity declined in control fruits at the beginning of stage II of fruit development and remained constant at the end of the experiment (Fig. 3B). In ED fruits, NADP-IDH was reduced gradually during fruit development. Overall early defoliation induced an increase in NADP-IDH activity during fruit development. A sharp rise of 53% of NADP-IDH was observed 1 week after defoliation in ED fruits, compared to the control, while no significant change was found 7 weeks after defoliation. The highest NADP-IDH activity was detected in ED fruit 48 h after defoliation (Fig. 3B).
The same pattern of GABA content was observed in control and ED plants during fruit development (Fig. 3C) where a gradual decline was observed with an increase in GABA content only at 127 days of fruit development. Early defoliation significantly affected GABA content and succinate semialdehyde dehydrogenase (SSADH) mRNA levels (Fig. 3C; 3D). A sharp drop in GABA content was found in the ED fruits, 48 h (29%) and 1 week (23%) after defoliation, in comparison with the controls (Fig. 3C). Seven weeks after defoliation, the ED fruits had higher GABA content than control fruits. The relative expression level of SSADH was 2.5-fold and 1.4-fold higher in ED fruits than controls, 48 h and 1 week after defoliation, respectively while a sharp drop (52%) was observed 7 weeks after defoliation (Fig. 3D).
3.4. Effects of early defoliation on proline synthesis pathways
Overall, proline content declined in control and ED fruits during fruit development (Fig. 4). The relative expression level of the mRNA encoding P5CS was greater in ED fruits than controls during the experiment, while a significant decline in proline content was observed in ED fruits only 48 h after defoliation (Fig. 4A; B).
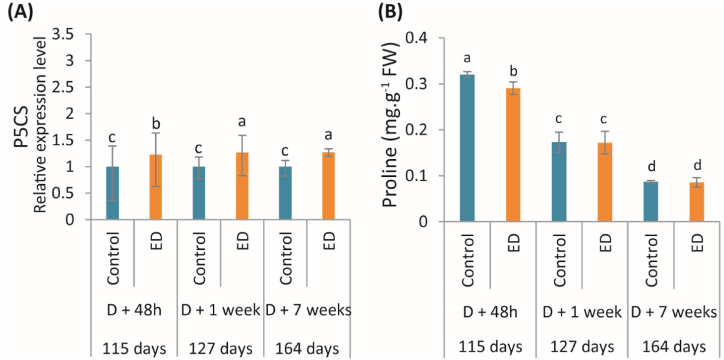
(A) Relative expression level of gene encoding Δ1-pyrroline-5-carboxylate synthase (P5CS), and (B) changes in proline content, measured at 48h, 1 week and 7 weeks following defoliation in fruits under control (blue bars) and early defoliation (ED) (orange bars) treatments. For each parameter, the corresponding period in the fruit growth is also given in days after anthesis. Data are mean values (±SD) of four independent measurements (n = 4). Different lowercase letters indicate significant differences between treatment and along the development stages (P < 0.05). (For interpretation of the references to color in this figure legend, the reader is referred to the Web version of this article.)
3.4.1. Effects of early defoliation on primary metabolism in the short- and long term
Hierarchical clustering with heat maps made it possible to separate the primary metabolism changes resulting from defoliation that make up the short- (48 h and 1 week following defoliation) and long-term response (7 weeks following defoliation) (Fig. 5). In addition, the clustering of metabolites and enzymes highlighted three main clusters. The first cluster (red cluster) gathered metabolites and enzymes involved in hexose and organic acid catabolic pathways, which were strengthened during the short-term defoliation response. This was illustrated by higher PFK, PEPC and NADP-IDH activities only 48 h after the early defoliation. Cluster 2 (orange cluster) grouped CsCit1 transcript and GABA content, which presented different pattern from the other clusters, with an increase 48 h and 1 week after defoliation. The pattern of the third cluster (yellow cluster) was clearly different from the others since it was characterized by enhanced levels of sucrose, hexose and citrate content during the long-term defoliation response (7 following defoliation). These patterns were also associated with increased acid INV activity, 48 h and 7 weeks after defoliation.
4. Discussion
In this study, we analyzed the metabolic changes occurring in mandarin fruit under partial defoliation-induced carbohydrate limitation during the second stage of fruit development. Partial defoliation is a well-known trigger for decreased respiration and rapid uptake of cellular carbohydrate content [32]. [24] showed significant transcriptional changes in berry plants after defoliation-induced modification of the source/sink ratio, affecting the composition of ripening fruits. Previous studies have reported that early defoliation induced significant alteration in citrus fruit including the decline of cell growth, sucrose, organic and amino acid content, mainly observed in stage III of fruit development [14]. Sucrose represents the main translocated carbohydrate and the major photo-assimilate stored in the plant [11]. In citrus fruit, roughly 50% of sucrose come from leaves photosynthesis during the period of fruit development [33], which means that other 50% of sucrose resulted in the metabolism of fruit itself. Our results show that sucrose and hexoses content were less affected by the early defoliation (Fig. 1) with concomitant increase in fruit growth [14]. These results suggested that defoliation induced in stage II of development can lead to an adaptive short-term response for carbohydrate partitioning in citrus fruits. In contrast, a decline in sugar content was observed in tomato [34], muskmelon [17] and mango [21] in response to carbohydrate limitation, and may be related to the time at which defoliation is applied during fruit growth. Among the enzymes involved in sucrose metabolism, invertase hydrolyzes sucrose to glucose and fructose, which contributes to the regulation of carbohydrates translocated into the sink tissues [35]. While acid invertase is mainly found in the vacuole and cell wall, neutral invertase is located in the cytoplasm [36]. In our study, acid invertase rapidly increased after early defoliation and was negatively correlated with sucrose and hexose content 48 h after defoliation (Fig. 5) suggesting the use of hexoses to meet the energy and substrate requirements for growth, storage and energy production. Resulting glucose and fructose were then catabolized in the cytosol. A previous study also reported that acid invertase activity is enhanced for hexose unloading out of the vacuole, during the second stage of development in tomato fruits subjected to source/sink ratio change via pruning [35]. Glycolysis is the main pathway catabolizing hexoses in the cytosol. In our study, both acid invertase and PFK activities were increased early in response to defoliation, i.e., 48 h after the ED, which suggested enhanced glycolytic flux to cope with carbohydrate limitation and recover energy for fruit growth (Fig. 1). It is worth to note that sucrose and hexoses content were sustained after ED despite the enhanced sucrose cleavage and glycolytic flux. We may assume that ATP-dissipating sucrose cycle (synthesis and degradation) could be optimized in order to avoid excess energy loss [37]. It has also been reported that slowing or suppressing futile cycles in central metabolism is a well-known strategy for enhancing both carbon and energy use efficiency [38]. In contrast, the long-term defoliation response (seven weeks after the ED) was characterized by enhanced acid invertase activity and mRNA expression level, and decreased PFK activity with a concomitant maintain of sucrose and hexoses content (Fig. 1E; 1F; Fig. 5). Thus, long-term defoliation response may imply a slowing glycolytic flux to allow a more efficient storage of hexoses in the vacuole. Taken together, our results indicated that the decreased source/sink ratio led to an adaptive short-term response (48h after the ED) resulting in an increased glycolytic flux to produce required energy and sustain respiration through the TCA cycle (Fig. 6).
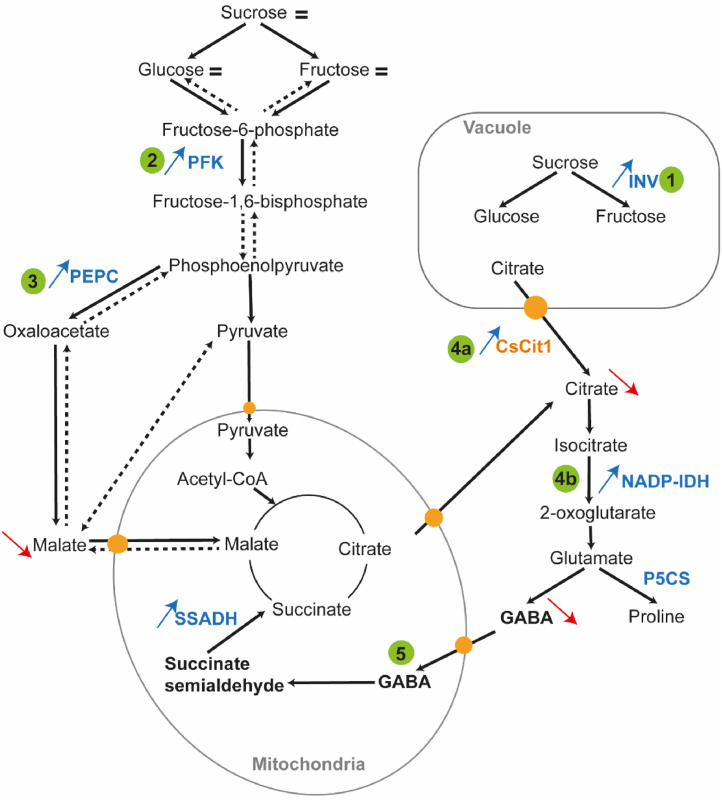
Proposed mechanism involved in short-term response (+48h) to the early defoliation in mandarin fruit based on q-PCR analyses of gene expression, enzymatic activities, and metabolite content. Sucrose and hexose content was near to control values despite the carbohydrate limitation. Partial defoliation leads to (1) vacuolar sucrose degradation, mediated by acid INV (acid invertase) enzyme associated with (2) enhanced glycolytic flux shown through increased PFK (phosphofructokinase) activity. (3) Malate synthesis mediated by PEPC (phosphoenolpyruvate carboxylase) is increased during carbohydrate limitation to maintain citrate synthesis via the TCA cycle. (4a) The high expression level of the transporter CsCit1 and (4b) enhanced NADP-IDH (NADP-isocitrate dehydrogenase) activity show the mobilization of citrate from the vacuole to the cytosol, where citrate is used to form glutamate. (5) The GABA shunt pathway seems to be increased during in response to early defoliation to feed the TCA cycle and sustain the respiration rate. Orange circles indicate the transporters, blue and red arrows indicate an increase and decrease in metabolite or enzyme 48h after the defoliation, respectively. Abbreviations: Acid INV, acid invertase, NADP-IDH, NADP-isocitrate dehydrogenase, P5CS, Δ1-pyrroline-5-carboxylate synthase; PFK, phosphofructokinase, SSADH, succinate semialdehyde dehydrogenase; TCA cycle, tricarboxylic acid. (For interpretation of the references to color in this figure legend, the reader is referred to the Web version of this article.)
Citrus fruits accumulate organic acids (mostly citrate and malate) during fruit development. A significant change in the total acidity occurs during the first half of stage II, due to the accumulation of citrate in the vacuole of juice sac cells, which then gradually decreases during second half of stage II and stage III [6]. Citrate is synthetized through the TCA cycle in the mitochondria. Firstly, oxaloacetate (OAA) and malate—intermediates of the TCA cycle—are synthesized by PEPC and NAD-malate dehydrogenase (NAD-MDH), respectively. Thus, both enzymes, with citrate synthase, are considered as promoting citrate biosynthesis [39]. In our study, the same trends in malate content and PEPC activity were found after early defoliation since it belonged to the same cluster (Fig. 5). Moreover, a decline in malate content was found during the experiment (Fig. 2A) [40]. also showed that partial defoliation induced a decrease in malate content in grape berries. Thus, malate could be converted into tricarboxylates, citrate essentially, through the TCA cycle in the mitochondria. The rapid increase in PEPC activity 48 h after defoliation could have stimulated glycolytic flux and helped to enhance OAA supply to the TCA cycle, which can offset citrate loss (Fig. 2C). However, PEPC activity dropped 1 and 7 weeks after defoliation, indicating a different PEPC contribution to sustaining high citrate content at the end of the experiment (Fig. 2; Fig. 5). Moreover [41], showed that among di- and tricarboxylic acids in the mitochondria, citrate is the main substrate for growth and respiration. Thus, a decrease in sucrose content due to partial defoliation induced a low respiration rate available for citrate synthesis. The citrate reduction observed in ED fruits may suggest it is being degraded in the cytosol, where it can be metabolized to isocitrate and 2-oxoglutarate by aconitase and NADP-IDH, respectively [42]. [43] showed the citrate reduction at the second fruit growth stage was related to activity of the H+/citrate symporter CsCit1. Our PCR data showed a rapid increase in CsCit1 mRNA levels in ED fruit, 48 h and 1 week after early defoliation, associated with the enhanced NADP-IDH activity (Fig. 3A; 3B), suggesting that citrate was released from vacuole and used in the cytosol in response to early defoliation. In the cytosol, the 2-oxoglutarate formed by NADP-IDH can be metabolized for amino acid production like glutamate [11]. Then, glutamate can be used for protein and amino acid synthesis, the largest consumer of amino acid in the early stage II of fruit development. In addition to protein synthesis, glutamate utilization can be induced by two pathways: the first involves its conversion into glutamine or proline and the second is related to its catabolism through the GABA shunt [6,12]. Proline is synthesized from the glutamate reduction by P5CS and can accumulate in many plant species in response to various environmental stresses, like high salinity, drought conditions and biotic stress [44]. In our study, proline content level decreased in ED fruits 48 h after the defoliation while it remained similar to control values one week and seven weeks after the defoliation. Although an increase in P5CS transcription level was reported, we may suggest that proline synthesis was not the main metabolic pathway involved in the fate of citrate in the cytosol (Fig. 4). Glutamate can also be catabolized in the cytosol through the GABA shunt. The latter is closely linked to the TCA cycle and consists of three reactions catalyzed by glutamate decarboxylase, GABA aminotransferase, and succinate semialdehyde dehydrogenase (SSADH) which catalyze the irreversible oxidation of succinic semialdehyde (SSA) to succinate, coupled with NADH production [45]. In our study, SSADH transcripts and NADP-IDH belonged to the same cluster (Fig. 5) and were enhanced 48 h after defoliation (Fig. 3B; 3D). These trends were associated with a decline in GABA content in ED fruits 48 h and 1 week after defoliation (Fig. 3C), suggesting the GABA shunt is involved in citrate degradation. Hierarchical clustering also grouped GABA content and CsCit1 transcript in the same cluster (Fig. 5), associated with the rapid increase in expression level of CsCit1 transcript after defoliation (Fig. 3A). These results illustrate the close connection between vacuolar citrate release and cytosolic degradation through the GABA shunt. Our results also suggested that GABA shunt may be the main supply to feed the TCA cycle and sustain respiration [46] during carbohydrate limitation (Fig. 5). This result is consistent with previous studies that reported the dependence of GABA biosynthesis on GAD protonation, and thus the cytosolic acidity induced by citrate release [45]. Increase in the cytosolic acidity was related to greater GAD activity and GABA biosynthesis in carrots [47] and tomatoes [48]. [12] also reported a similar mechanism in Citrus fruit for GABA shunt after citrate release from the vacuole. These results can be related to the findings of [39] who showed that the reduced vacuolar uptake of citrate leads to the higher expression of genes associated with GABA biosynthesis. Taken together, our results suggested that the mobilization of stored vacuolar metabolites and GABA shunt were the main pathway involved in the short-term defoliation response to sustain sucrose and hexoses content and respiration in citrus fruits (Fig. 6).
Moreover, it has been shown that the GABA shunt pathway may be induced by various environmental constraints and involved in oxidative stress [49]. The increase in reactive oxygen species production due to carbohydrate limitation was also suggested by transcriptome profiling analysis that found the activation of oxidative stress genes, such as catalase [50]. Thus, carbohydrate limitation can induce oxidative stress in Citrus, and as consequence, stimulate the GABA shunt pathway [51]. Some studies also have demonstrated involvement of the GABA shunt in plants exposed to different environmental stresses [[52], [53], [54]].
5. Conclusion
In this study, we showed that partial defoliation during early growth induced a short adaptive response to maintain sucrose and hexoses content. This metabolic fit involved slowing sucrose cycle, high glycolytic flux and citric acid mobilization through the GABA shunt pathway. We also reported that vacuolar storage and cytosolic degradation were strongly interrelated in low source/sink ratio conditions. GABA shunt appeared to be the main citrate degradation pathway to feed the TCA cycle and sustain respiration rate in response to early defoliation. These results demonstrated that citrus fruits can rapidly respond to carbohydrate limitation, which can alter the fruit quality. Further studies will focus on the effect of partial defoliation at the end of stage II of development, when greater carbon needs are required to sustain high respiration rate for fruit growth. Management of fruit acidity is one of the main research areas for improving citrus fruit quality. Thus, knowing the response to an environmental factor that strongly reduces carbohydrate assimilation in fruit and its adaptative mechanisms is important from both a fundamental and agronomic viewpoint.
Author contribution statement
Radia Lourkisti; Jérémie Santini: Analyzed and interpreted the data; Wrote the paper.
Sandrine Antoine: Performed the experiment.
Olivier Pailly; François Luro; Yves Gibon; Julie Oustric: Contributed reagents, materials, analysis tools or data.
Liliane Berti: Conceived and designed the experiments; Analyzed and interpreted the data; Wrote the paper.
Data availability statement
Data included in article/supp. material/referenced in article.
Declaration of interests
The authors declare no competing interests.
Acknowledgments
This work was supported by the "Collectivité de Corse".
References
Articles from Heliyon are provided here courtesy of Elsevier
Full text links
Read article at publisher's site: https://doi.org/10.1016/j.heliyon.2023.e15573
Read article for free, from open access legal sources, via Unpaywall:
http://www.cell.com/article/S2405844023027809/pdf
HAL Open Archive
https://hal.inrae.fr/hal-04176065
Similar Articles
To arrive at the top five similar articles we use a word-weighted algorithm to compare words from the Title and Abstract of each citation.
Short- and long-term effects of carbohydrate limitation on sugar and organic acid accumulation during mandarin fruit growth.
J Sci Food Agric, 96(11):3906-3914, 08 Feb 2016
Cited by: 3 articles | PMID: 26694637
Comparative transcriptome analysis reveals key genes potentially related to organic acid and sugar accumulation in loquat.
PLoS One, 16(4):e0238873, 29 Apr 2021
Cited by: 9 articles | PMID: 33914776 | PMCID: PMC8084190
Inhibition of aconitase in citrus fruit callus results in a metabolic shift towards amino acid biosynthesis.
Planta, 234(3):501-513, 28 Apr 2011
Cited by: 35 articles | PMID: 21528417
How and why does tomato accumulate a large amount of GABA in the fruit?
Front Plant Sci, 6:612, 10 Aug 2015
Cited by: 38 articles | PMID: 26322056 | PMCID: PMC4530592
Review Free full text in Europe PMC