Abstract
Free full text

Suspension Matrices for Improved Schwann-Cell Survival after Implantation into the Injured Rat Spinal Cord
Abstract
Trauma to the spinal cord produces endogenously irreversible tissue and functional loss, requiring the application of therapeutic approaches to achieve meaningful restoration. Cellular strategies, in particular Schwann-cell implantation, have shown promise in overcoming many of the obstacles facing successful repair of the injured spinal cord. Here, we show that the implantation of Schwann cells as cell suspensions with in-situ gelling laminin:collagen matrices after spinal-cord contusion significantly enhances long-term cell survival but not proliferation, as well as improves graft vascularization and the degree of axonal in-growth over the standard implantation vehicle, minimal media. The use of a matrix to suspend cells prior to implantation should be an important consideration for achieving improved survival and effectiveness of cellular therapies for future clinical application.
Introduction
Spinal-cord trauma produces deficits in motor, sensory, and autonomic function as a result of extensive cell death, axonal disruption, and demyelination (Profyris et al., 2004). Following injury, the limited inherent capacity for repair possessed by the central nervous system (CNS) means that therapeutic measures must be undertaken to compensate for this deficiency (Pearse and Barakat, 2006; Pearse and Bunge, 2006). The implantation of exogenous cells, derived from a variety of sources, has been demonstrated to be an effective approach for overcoming many of the barriers to successful spinal-cord repair, as well as promoting improved functional outcome (Franssen et al., 2007; Louro and Pearse, 2008; Nandoe Tewarie et al., 2007; Pearse and Barakat, 2006)
The implantation of Schwann cells (SCs), the myelinating glia of the peripheral nervous system (PNS) important for facilitating regeneration after nerve injury, has been shown to provide neuroprotection (Pearse et al., 2004; Schaal et al., 2007; Takami et al., 2002), support axonal regrowth and remyelination, (Bunge and Pearse, 2003; Pearse et al., 2004, 2007), as well as improve functional outcome in experimental models of both acute (Pearse et al., 2004, 2007) and chronic spinal cord injury (SCI) (Barakat et al., 2005). Their reparative efficacy, however, may be limited by their survival rate post implantation; approximately 20% of grafted SCs survive within the subacutely (Hill et al., 2007; Pearse et al., 2007) or chronically (Barakat et al., 2005) contused spinal cord. The dramatic loss of implanted SCs via necrotic and apoptotic cell death occurs largely during the first 3 weeks following implantation (Hill et al., 2007; Pearse et al., 2007), and may be attributed to both the implantation environment, with low oxygen levels, oxidative metabolites, inflammatory cytokines, and a cell-mediated immune response (Hill et al., 2007; Pearse et al., 2007), and/or to the withdrawal of trophic/mitogenic factors and adequate extracellular matrix adherence, termed anoikis, when taken from their pre-implantation culture conditions (Frisch and Screaton, 2001).
In the current study, we investigated whether implantation of SCs within various injectable, in-situ gelling matrix formulations as suspension grafts would improve their long-term survival within the contused, adult thoracic spinal cord, as opposed to a previously employed DMEM/F12 media suspension. Although the use of SC-seeded matrices has been a fundamental component of bridging strategies for peripheral nerve injury (Johnson et al., 2005) and following complete spinal-cord transection (Fouad et al., 2005; Meijs et al., 2004; Xu et al., 1997), their use in more clinically relevant SCI models has been largely unexplored. In addition, studies to compare the utility of different matrices in supporting implanted cell survival and/or enhancing their ability to act as a substrate for axon growth or for promoting revascularization following contusive SCI, to our knowledge, has not been undertaken.
The matrices used for these investigations were chosen based upon: (a) their successful and independent use as bridging scaffolds within nervous-system injury models (Bunge and Pearse, 2003; Iannotti et al., 2003); (b) known physical properties that allow them to provide a fluid suspension with cells for controlled injection directly into the injured CNS (Feng et al., 2005; Iannotti et al., 2003; Stabenfeldt et al., 2006); and (c) their adhesive properties for SCs (Armstrong et al., 2007). Fulfilling these criteria were the following matrices: (a) methylcellulose (Sigma-Aldrich), a biopolymer that is a methyl ether of cellulose, which has been shown to be an effective material for neural tissue-engineering therapies, mainly in the area of drug or molecular, rather than cellular, delivery (Tate et al., 2001); (b) extracellular matrix (ECM; Sigma-Aldrich) gel composed of laminin and collagen type IV, both of which have been demonstrated to be supportive matrices for SCs (Kassar-Duchossoy et al., 2001; Pierucci et al., 2008; Stabenfeldt et al., 2006); and lastly (c) Matrigel™ (BD), another matrix composition of laminin and collagen type IV, with molecules including heparan sulfate proteoglycans, entacin, and growth factors. BD Matrigel is derived from a gelatinous protein mixture secreted by mouse tumor cells, and has been employed conjunctively with SC grafting in models of spinal-cord hemisection (Iannotti et al., 2003) and complete transection (Fouad et al., 2005; Meijs et al., 2004; Xu et al., 1997). In these studies, the SC:BD Matrigel mix was placed within a biopolymer channel and used to bridge the injury site so as to provide a substrate for axon regeneration. In the current study, the three matrices or DMEM-F12 media were mixed with a defined number of enhanced green fluorescent protein(EGFP)-labeled SCs (2×
106 cells), and implanted directly into the epicenter of the injured adult rat thoracic spinal cord at 1 week following a moderate contusion.
Methods
Cultures
Schwann cells
SCs were obtained from the sciatic nerves of adult female Fischer rats, as previously described (Morrissey et al., 1991). SCs were then purified and expanded in accordance with published methods (Meijs et al., 2004). The cells were grown to confluency, and passaged to new dishes three times (P3) prior to transplantation. Their purity for grafting, using a method described earlier (Takami et al., 2002), was determined to be 95–98%.
Lentiviral vectors
Lentiviral-vector preparation
A lentiviral vector encoding enhanced GFP (EGFP) was used to transduce the SCs at passage one, so as to enable their long-term in-vivo tracking (Pearse et al., 2007). Viral-vector preparation was performed as previously described by Follenzi and colleagues (2000). Briefly, the gene coding for EGFP was subcloned into a lentiviral-vector plasmid. This plasmid contained the cytomegalovirus (CMV) promoter, which drives transgene expression, and the Woodchuck post-transcriptional regulatory element (WPRE), which improves mRNA transport. Cultured 293T cells were used for transfection of plasmids and viral harvesting. Prior to being resuspended in phosphate buffered saline (PBS), the virus was concentrated by ultracentrifugation at 20,000g. The viral vectors were then titered for transducing units either on 293T cells or by using an enzyme-linked immunosorbent assay (ELISA; Perkin Elmer) for quantifying p24 core protein concentrations, according to the manufacturer's instructions. Purified viral vector stocks were stored at −80°C until SC infection.
Lentiviral-vector transduction of Schwann cells to express enhanced green fluorescent protein
Lentiviral vector encoding EGFP was used to infect SCs at passage one. Cultures were infected by the addition of a predetermined volume of the concentrated virus in DMEM with 10% FBS and mitogens. A multiplicity of infection (MOI) of 50 was used to ensure high transduction efficiency (>95%) with an absence of toxicity.
Animals
Adult female Fischer rats (n=
51, 180–200
g) were housed in accordance with NIH guidelines and The Guide for the Care and Use of Animals. The Institutional Animal Care and Use Committee of the University of Miami approved all animal procedures. Prior to spinal-cord surgery, the rats were anesthetized (45
mg/kg ketamine, 5
mg/kg xylazine) by intraperitoneal injection.
Moderate thoracic contusion injury
A moderate thoracic (T8) contusion injury was induced using the MASCIS weight-drop device developed at New York University (Gruner, 1992). Prior to injury, a laminectomy was performed at thoracic vertebra T8. The laminectomy exposed the dorsal surface of the spinal cord underneath (T9) without disrupting the dura mater. A moderate injury to the spinal cord was enacted by dropping a 10-g weight from a height of either 12.5mm (histological studies; n
=
37) or 25.0
mm (behavioral studies; n
=
14). Animals (n
=
7) were excluded immediately when the height or velocity errors exceeded 7% or if the compression distance was not within range (Barakat et al., 2005; Pearse et al., 2007). Following injury, the overlying musculature was sutured and the skin closed using wound clips. The rats were allowed to recover in a warmed cage with water and food easily accessible. The rats received post-operative treatment and care that included the administration of Gentamicin (5
mg/kg, intramuscular; Abbott Laboratories), Buprenex (0.3
mg/kg, subcutaneous; Reckitt Benckiser), and lactated ringers, as described elsewhere (Pearse et al., 2007).
Schwann-cell implantation
Prior to implantation, EGFP transduced SCs were harvested from culture via trypsinization, then centrifuged, resuspended, and counted. Subsequent to counting, SCs were resuspended as aliquots in DMEM/F12 medium and were kept on ice prior to surgery. For SC implantation, 1 week post injury, rats were reanesthetized with 2% halothane, and the injury site was exposed. For the histological studies, animals were randomly assigned to one of four treatment groups, receiving the EGFP-SC suspensions of 2×
106 cells within DMEM/F12 medium (n
=
9, Hyclone; 8
μl total volume) or a specific fluid matrix: methylcellulose (n
=
8, Sigma-Aldrich), ECM gel (n
=
9, Sigma-Aldrich), or Matrigel (n
=
9, BD). For the non-medium groups, DMEM/F12 media was removed immediately prior to implantation, and SCs were resuspended in the specific matrix to be tested. Cells were implanted into the injury epicenter at a depth of 1
mm using a 10-μl siliconized Hamilton syringe with a pulled, beveled glass-pipette tip (100
μm diameter) held in a micromanipulator with a microinjector at a rate of 2
μl/min (World Precision Instruments). The injection pipette was kept in place for an additional 3
min to minimize leakage upon withdrawal. For the behavioral cohort, animals received EGFP-SCs within either DMEM/F12 medium (n
=
6) or Matrigel (n
=
8) in accordance with these same procedures. Following SC implantation, the muscle layers and the skin were closed separately, and animals received the aforementioned post-operative care. ECM gel and BD Matrigel were used without any additional modification of the supplier's product, and were kept on ice until SC suspension and injection. For the methylcellulose group, a 5% methylcellulose in D-PBS solution was prepared under aseptic conditions, in accordance with previous methodology (Tate et al., 2001), and kept at room temperature until use.
Histology
At 10 or 13 weeks post injury (9 or 12 weeks post transplantation; behavior or histology groups respectively), the rats were anesthetized (70mg/kg ketamine, 10
mg/kg xylazine) and transcardially perfused with 4% paraformaldehyde (PFA, 0.1M, pH 7.4) (Pearse et al., 2001). The brain and spinal cord were removed and post-fixed overnight in 4% PFA at 4°C and then cryoprotected via transfer to phosphate-buffered 30% sucrose at 4°C. The T7-11 thoracic spinal cords (20-mm-long piece), encompassing the lesion and graft site, were then sectioned on a cryostat at 20
μm, and mounted in five series onto Snowcoat X-tra slides (Surgipath Medical Ind., Inc.).
Immunohistochemistry
Four series were immunochemically stained for fluorescent microscopy with specific antibodies, as previously described (Pearse et al., 2007), while keeping the spontaneous fluorescence of the EGFP labeled SCs intact. The first series was used for staining host astrocytes using a GFAP antibody (1:1000; DAKO) to define the graft:host border and permit stereological quantification of SC survival. The second, third, and fourth series were used for staining axons, blood vessels, or proliferating cells using polyclonal pan-neurofilament (NF-H and NF-M and NF-L, 1:500; Encor Biotechnology), laminin (1:1000; Sigma-Aldrich), or Ki-67 (1:100; Abcam) antibodies respectively. After the primary antibodies were added, the tissue was incubated with an appropriate fluorescent secondary antibody, Alexa 594-conjugated goat anti-rabbit (1:200; Molecular Probes). Sections were then cover-slipped with Vectashield mounting medium (Vector Laboratories) containing the nuclear dye Hoechst and kept at 4°C.
Stereological quantification
Stereological quantification of EGFP-SC survival and proliferation (double labeling with Ki-67) was conducted throughout the volume of the 20-mm length of the T7-T11 spinal cord using unbiased computer-assisted microscopy and StereoInvestigator 3.0 software (MicroBrightField). The area of interest was first traced with the 20×
objective and logged into the StereoInvestigator software via the serial section manager. The optical fractionator then divided the area into 50
×
50
μm2 counting frames and automatically determined the appropriate grid size to allow 35 sampling sites within this area for each section. In each sampling site, the number of SCs was marked and recorded using a dissector probe under a 63
×
objective. Algorithms for estimating the total counts within the area and then volume were performed according to the StereoInvestigator software (Pearse et al., 2007). Approximately 10 to 12 sections across the entire width of the spinal cord (200-μm intervals) were used for this quantitative assessment of cell numbers stereologically. Axon and blood-vessel areas were measured within EGFP-SC grafts using Metamorph Imaging software (Molecular Devices), as previously described (Pearse et al., 2004). In brief, three sections from the center of the SC graft from each animal were imaged on an Olympus BX51 microscope (Olympus America Inc.), the fluorescent intensity of each image after conversion to black and white was then thresholded to remove the background and obtain only filamentous immunoreactivity. The thresholded area of positive staining was then calculated and averaged across the three sections using Metamorph (Molecular Devices).
Behavioral testing
Evaluation of gross locomotor performance in the open field was determined according to the Basso, Beattie, and Bresnahan (BBB) scale developed by Basso and colleagues (1995). Testing occurred weekly from 1 to 9 weeks post implantation by two observers blinded to the studies' group allocation. In addition, a BBB subscore was employed to provide a separate assessment of hindlimb and tail positioning, as described elsewhere (Pearse et al., 2007). Deficits in descending motor control were evaluated by testing the animals on an irregularly spaced grid-walk apparatus pre-injury (baseline) and at 2, 4, 6, and 8 weeks post injury, as previously described (Pearse et al., 2004).
Statistical analysis
For statistical analysis of data sets, a one-way analysis of variance (ANOVA) followed by the Tukey post-test were used to compare the rates of SC survival and proliferation, as well as the degree of axon and blood-vessel support provided by the different cell-seeded matrices versus DMEM/F12 medium controls. A repeated-measures ANOVA with a Bonferroni post-test was used to analyze temporal differences in functional recovery between medium and matrix EGFP-SC groups from 1 to 8 weeks post implantation. Significant differences were accepted at p<
0.05, p
<
0.01, and p
<
0.001. All errors are provided as standard errors of the mean. For independent linear correlation analyses between SC survival rates and altered axonal or vascular components of the graft by expression of Pearson correlation coefficients (R), standard R tables were employed to calculate statistical significance from degrees of freedom (n
−
2) (Pearse et al., 2005). Significant differences were accepted at the values listed previously.
Results
Schwann-cell morphology and migration were largely unaffected by matrix suspension
The implanted SCs, as described previously (Pearse et al., 2007), remained confined to the lesion site, encased strongly by GFAP immunoreactive host astrocytes; the host cord restriction of exogenous SC migration was not relieved by their grafting in different matrices (Fig. 1A–D). Evidence of the matrices into which the SCs were grafted was not apparent in either the ECM gel or BD Matrigel groups, although the presence of clear regions, devoid of cellular infiltrate within only those animals receiving methylcellulose, may have indicated that this material had not fully degraded following implantation. The morphology of EGFP-SCs within the lesion environment was largely similar among the grafted groups, with SCs exhibiting a characteristic spindle shape with a narrow cell body and very long processes (Barakat et al., 2005; Pearse et al., 2007). Lastly, those animals receiving SCs implanted within laminin:collagen matrices displayed a very pronounced swirling pattern within the implant site (Fig. 1B,C) that was not evident in the other groups and was similar to what is observed when they are in culture (Pearse et al., 2007). This could indicate that the cells were mitotically active, although no distinction in the number of proliferating SCs was observed among the groups (see below).
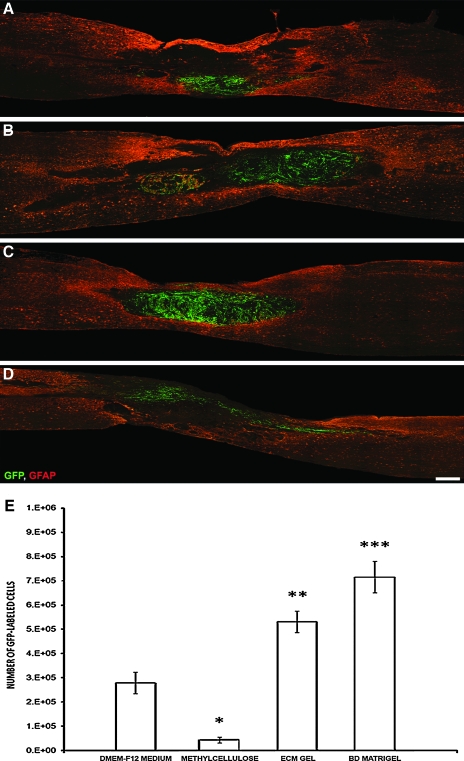
BD Matrigel and ECM gel, but not methylcellulose, improve GFP-SC numbers over cell:medium suspensions (A–D). A significant improvement in SC numbers at 12 weeks following implantation into the injured spinal cord is achieved over DMEM-F12 medium suspensions (A) when the cells are injected within ECM gel (B) or BD Matrigel (C) but not methylcellulose (D). Sections are 20-μm thick sagittal sections taken from the center of the SC graft from an animal with the overall SC survival rate closest to that of the mean for that group. SCs are labeled with GFP (green) and host astrocytes are stained for GFAP (red). Scale bar: 500μm. (E) Stereological quantification of SC numbers post implantation shows a significant increase when laminin:collagen matrices are employed; a significant decrease was observed compared to medium controls when methylcellulose was used. Statistical significance indicated at *p
<
0.05, **p
<
0.01, or ***p
<
0.001. Color image is available online at www.liebertonline.com/neu.
Schwann-cell numbers were enhanced within laminin: Collagen matrices
Stereological quantification of EGFP-SC numbers at 12 weeks post implantation revealed that, of the original 2 million cells injected into the injured spinal cord, 279,200±
44,300 (or 14%) were present following their suspension in DMEM-F12 medium (Fig. 1A,E). The survival rate is in accordance with earlier reports (Hill et al., 2007; Pearse et al., 2007). A small percentage of the cells may have also been lost during the ex-vivo preparation and injection procedures (Hill et al., 2007). There was a 190% increase in SC numbers with ECM gel (531,300
±
44,100; an overall survival rate of 27%, t34
=
5.478, p
<
0.01; Fig. 1B,E), and a 256% increase with BD Matrigel (715,900
±
64,800; an overall survival rate of 36%, t34
=
9.491, p
<
0.001; Fig. 1C,E). In contrast, suspension in methylcellulose not only failed to enhance SC survival but actual significantly reduced numbers by 85.4% compared to medium suspension (43,500
±
11,300; an overall survival rate of 2%, t34
=
4.970, p
<
0.01; Fig. 1D,E). BD Matrigel also proved to improve SC numbers significantly compared to ECM gel (t34
=
4.013, p
<
0.05).
To identify proliferating EGFP SCs within the lesion/implant site, we looked for co-labeling of the cell proliferation marker Ki-67, a protein present only during active phases of the cell cycle (Scholzen and Gerdes, 2000), within GFP fluorescing SCs (Fig. 2A–C) at the study endpoint. We found that, irrespective of implant group, approximately 3–5% of implanted SCs were Ki-67 positive (Fig. 2D). These cells were located predominately on the outer fringes of the implant.
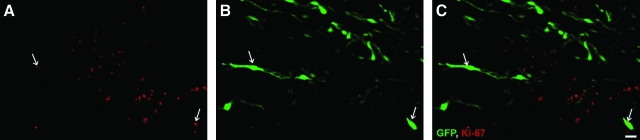
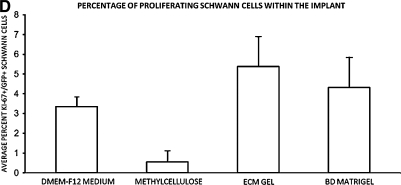
Proliferation of GFP-SCs are unchanged by suspension vehicle (A–B). Immunochemical staining with the cell proliferation marker Ki-67 (A) of GFP-SC implants (B) shows that at 12 weeks post implantation only a small percentage of cells are undergoing cell division; these cells are localized predominantly to the outer fringes of the implant (C, white arrows indicate co-localization). Scale bar: 20μm. (D) No significant difference in the percentage of GFP-SCs undergoing proliferation was observed among matrix and medium groups. Color image is available online at www.liebertonline.com/neu.
Laminin: Collagen matrices containing Schwann cells exhibit greater revascularization and axonal in-growth
Evaluation of the SC graft/lesion site also demonstrated significant disparity among the groups in the amount of revascularization and axonal in-growth. To determine the degree of graft vascularization, areas of intensely immunoreactive, filamentous laminin staining, indicative of blood vessels, was quantified. The average area covered by blood vessels within SC grafts containing DMEM-F12 media was 0.65±
0.13
mm2 (Fig. 3A,E). Suspension grafts composed of methylcellulose displayed few blood vessels (Fig. 3D), an 85% reduction compared to medium controls (t25
=
4.623, p
<
0.05; Fig 3E), due to the dramatically smaller implant sizes. Vascularization of the ECM gel and Matrigel SC suspension grafts (Fig. 3B,D) was significantly greater than that of DMEM controls, with increases of 131% and 134%, (t25
=
7.370, t25
=
7.261, p
<
0.001 for both matrices respectively; Fig. 3E).
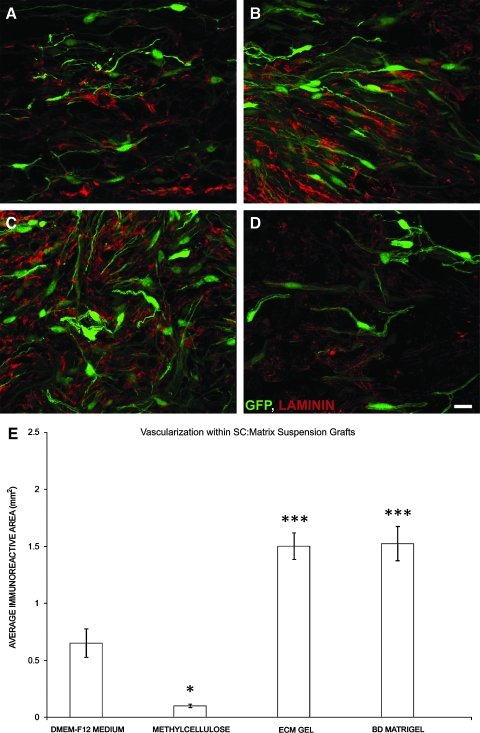
BD Matrigel and ECM gel, but not methylcellulose, improve SC implant vascularization over cell:medium suspensions (A–D). To determine the degree of GFP-SC implant vascularization, immunostaining for laminin (red) was performed, and the area of immunoreactivity within the implant quantified. Compared to medium (A), suspension of GFP-SCs in either ECM gel (B) or BD Matrigel (C), but not methylcellulose (D), significantly increased the amount of filamentous laminin within the implant. Scale bar: 20μm. (E) Quantification of the area of filamentous laminin within the implants revealed greater vascularization with ECM gel and BD Matrigel over medium controls; significantly less vascularization was observed with methylcellulose suspension. Statistical significance indicated at *p
<
0.05 or ***p
<
0.001. Color image is available online at www.liebertonline.com/neu.
The degree of axonal in-growth into the SC grafts, composed of different matrices, mirrored that of their vascularization, indicating a close relationship between these events. In SC:DMEM-F12 medium suspension implants (Fig. 4A), the average area of neurofilament axon immunoreactivity, as a measure of axonal in-growth, was determined to be 1.03±
0.18
mm2 (Fig. 3E). With poor support for SC survival, grafts containing methylcellulose exhibited a significant reduction (92%) in axonal growth compared to medium controls (t25
=
7.205, p
<
0.001; Fig. 4D,E). On the other hand, axon in-growth was significantly enhanced within suspension implants of ECM gel (79%, t25
=
6.406, p
<
0.001; Fig. 4B,E) or BD Matrigel (97%, t25
=
7.589, p
<
0.001; Fig. 4C,E).
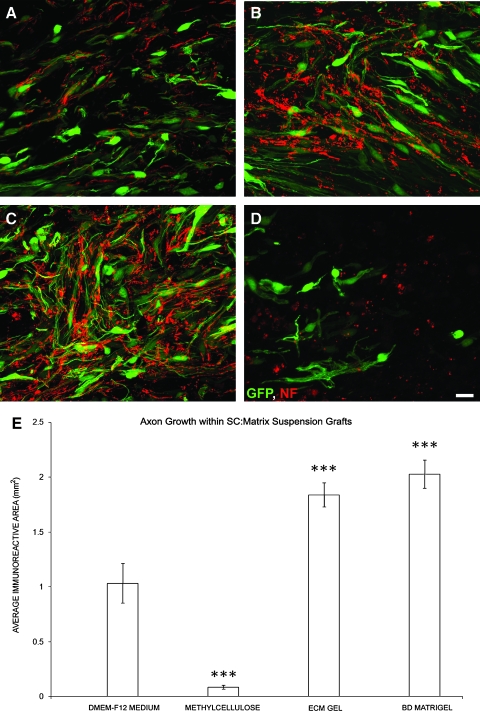
BD Matrigel and ECM gel, but not methylcellulose, improve axonal in-growth within the SC implant over cell:medium suspensions (A–D). To determine the degree of axonal in-growth within the GFP-SC implants, we employed immunostaining for neurofilaments (red) and subsequent quantification of the area of immunoreactivity within the implant. Compared to medium (A), suspension of GFP-SCs in either ECM gel (B) or BD Matrigel (C), but not methylcellulose (D), significantly increased the degree of axonal in-growth within the implant. Scale bar: 20μm. (E) Quantification of the area of neurofilament immunoreactivity within the implants revealed greater axonal in-growth with ECM gel and BD Matrigel over medium controls; significantly less axonal in-growth was observed with methylcellulose suspension. Statistical significance indicated at ***p
<
0.001. Color image is available online at www.liebertonline.com/neu.
Revascularization and axonal in-growth within Schwann-cell implants strongly correlates to Schwann-cell numbers
To examine the relationship between SC numbers and the degree of graft vascularization or axonal in-growth, we performed linear correlation analyses using the expression of Pearson correlation coefficients (R). These coefficients were obtained from data plots of these variables, SC numbers versus either blood vessel (Fig. 5A) or axon areas (Fig. 5B), from individual animals across implant cohorts. We found that a significant relationship existed between both the amount of graft vascularization (R=
0.687, p
<
0.01) or axonal in-growth (R
=
0.712, p
<
0.01) and the numbers of EGFP-SCs present within the implant/lesion site. This implies that SC survival and/or proliferation required good vascularization of the graft and axonal ingress, which had been supported by the matrix into which the SCs were suspended prior to implantation.
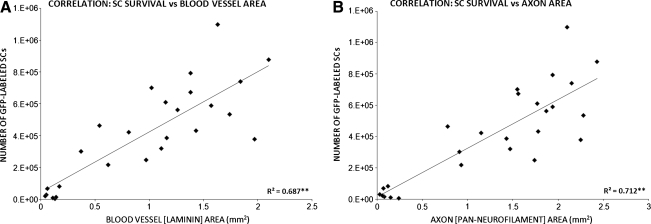
Numbers of GFP-SCs within the injured spinal cord correlates with the degree of implant vascularization and the amount of axonal in-growth (A–B). Linear correlation analysis of animals from all implant groups indicated a significant relationship between SC numbers and both graft vascularization (A) and axonal in-growth (B). Statistical significance indicated at **p<
0.01.
Suspension of Schwann cells in Matrigel leads to improved locomotor recovery after spinal cord injury compared to when they are implanted in medium
No significant difference in locomotor performance in the open field, as measured using the BBB score, was observed between SC-Matrigel and SC-medium groups during the first 4 weeks post injury (4 weeks: 9.9±
0.3 vs. 9.8
±
0.5 respectively, p
>
0.05; Fig. 6A). However, beginning at 5 weeks post SCI (4 weeks post implantation) and continuing through to the endpoint, animals receiving SC-Matrigel implants performed significantly better in the open field than their SC-medium counterparts (9 weeks: 11.1
±
0.3 vs. 9.8
±
0.4 respectively, t12
=
3.161, p
<
0.01; Fig. 6A). Subsequent analysis of BBB subscores, however, revealed no differences in hindpaw positioning between the two SC-implanted groups during the post-injury period (9 weeks: 1.1
±
0.3 vs. 0.7
±
0.2 respectively, p
>
0.05; Fig. 6B). Evaluation of hindpaw placement on an irregularly spaced grid-walk test revealed a significant deficit (number of footfall errors) at 1 week post SCI compared to uninjured baseline values (8.5
±
1.1 vs. 0.3
±
0.1 errors respectively, t12
=
3.992, p
<
0.001). Although no significant difference in grid-walk ability between SC-medium and SC-Matrigel groups was observed at 2 weeks post injury, at 4 weeks, animals receiving SC-Matrigel implants exhibited significantly fewer footfall errors than those treated with SC medium (5.8
±
0.5 vs. 7.8
±
0.6 errors respectively, t12
=
2.682, p
=
0.015). At 8 weeks after SCI, however, no difference in footfall errors between the SC-implanted groups was observed.
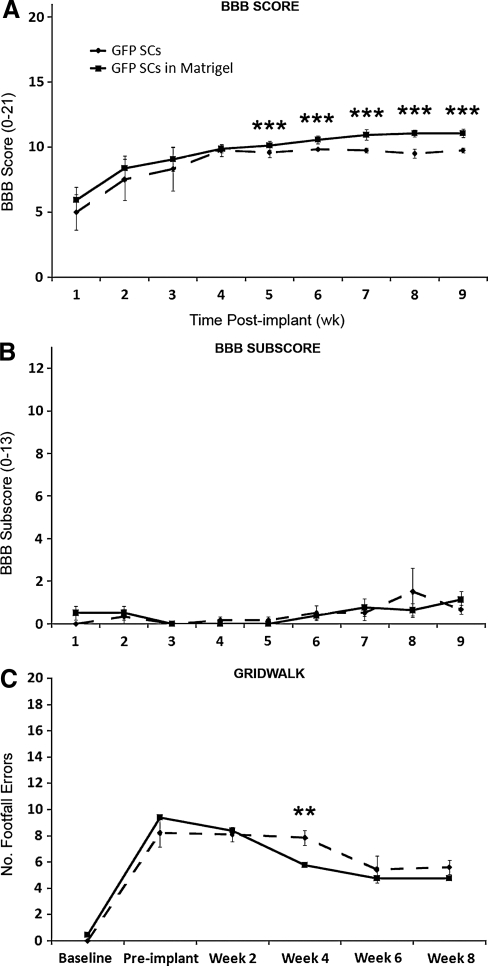
Suspension of GFP-SCs in BD Matrigel led to improved hindlimb function compared to SC-medium controls. BBB locomotor testing revealed a significant improvement with SC-BD Matrigel over SC-medium implants from 4 weeks post implantation (A), although no improvement was observed in subsequent BBB subscore analysis (B). Foot positioning on the grid-walk test showed a transient improvement with BD Matrigel at 4 weeks post implantation compared to medium controls, but this did not persist through 6–8 weeks (C).
Discussion
The current study evaluated several in-situ gelling matrices (synthetic as well as ECM) for their ability to support implanted SC survival after SCI and to enhance anatomical and functional outcomes over standard SC-medium suspensions. Although previous work has demonstrated that SC-medium implantation leads to spinal-cord repair and behavioral recovery in acute and chronic experimental SCI models (Barakat et al., 2005; Pearse et al., 2004, 2007; Takami et al., 2002), significant cell loss (~80%) may limit their effectiveness (Barakat et al., 2005; Hill et al., 2007; Pearse et al., 2007). We have shown in this investigation that the suspension of SCs in matrices composed of laminin and collagen significantly increases SC survival, graft vascularization, and axonal in-growth over medium controls. Through the use of independent linear correlation analyses, both blood vessel and axonal density were demonstrated to correlate strongly with SC numbers, indicating that improved implant vascularization and axonal support within the matrix may have provided a more supportive environment for the implanted SCs. Moreover, the suspension of SCs in Matrigel significantly enhanced gross locomotor function, as assessed using the BBB score, over animals that received SC medium. In contrast, the use of a synthetic matrix, methylcellulose, reduced SC numbers, as well as blood-vessel and axonal density within the implants, compared to SC medium, indicating that specific properties are required of the in-situ gelling matrices for these beneficial effects.
Previous studies by our group and others have shown that ~15–20% of SCs, when suspended within minimal culture medium, survive following their implantation into the injured spinal cord (Barakat et al., 2005; Hill et al., 2007; Pearse et al., 2007). This dramatic cell loss could be caused by a lack of adequate support, including serum-mitogen withdrawal, oxygen starvation, and the disruption of cell adhesions, as well as a hostile implant environment composed of oxidative metabolites, proinflammatory cytokines, and tissue-degrading proteases (Bao and Liu, 2002; Brundin et al., 2000; Hall and Braughler, 1986; Pearse et al., 2003). In the present study, the use of laminin:collagen matrices, Matrigel or ECM gel, improved implanted SC numbers approximately twofold. ECM proteins like collagen and laminin can regulate SC proliferation and survival (Armstrong et al., 2007), as well as promote effective cellular adhesion and spreading by modulating their actin cytoskeleton dynamics (Sugrue and Hay, 1986). These ECM proteins possess an arginine–glycine–aspartic acid sequence (RGD) that is recognized by integrins, a family of cell surface receptors that mediate cell–cell and cell–ECM adhesion (McKerracher et al., 1996). ECM binding thus allows integrins to regulate, through altered GTPase activity (Relvas et al., 2001), a broad range of cellular processes (Kariya et al., 2004; Kim, 2000; Stupack, 2005). In the case of SCs, ECM binding also serves as a prerequisite to myelin formation (Podratz et al., 2001).
Laminin, an integral part of the basement membrane, governs many important SC functions (Chen and Strickland, 2003), including their migration, differentiation, and axon association, and is the major ECM produced by SCs (Cornbrooks and Bunge, 1983). The environment provided by scaffolds composed of laminin have been demonstrated not only to increase SC proliferation in culture but have also been shown to be critical to basement membrane formation following SC implantation into the injured peripheral nervous system (Armstrong et al., 2007), providing an adherent substrate for both SCs and in-growing axons. SC adherence to laminin within the hostile environment of the injured spinal cord may not only activate important cell survival and proliferation pathways and provide a protective niche for the implanted cells, but laminin could also prevent anoikis, a process whereby the cells undergo apoptosis due to a lack of adequate adhesion (Frisch and Screaton, 2001). Many cell types require anchorage to ECM within tissues to maintain their growth and survival (Frisch and Ruoslahti, 1997).
Likewise, collagen has proven to be an effective ECM for SC adhesion because of its ability to promote SC process extension and their association with axons for ensuring myelination (Chernousov et al., 2008). Collagen's properties give it high mechanical strength, significant permeability and water-uptake properties, exceptional biocompatibility and biodegradability, as well as low antigenicity (Han et al., 2009; Tabesh et al., 2009). Collagen therefore has served as an attractive matrix for tissue engineering because of these properties and its functional role in connective-tissue formation, as well as its critical importance in wound healing, being a potent substrate for angiogenesis and the revascularization of injured tissues (Serini et al., 2006). Collagen can also bind various molecules, including neurotrophic factors that are in addition supportive for cell migration and axon extension (Han et al., 2009).
The use of Matrigel, which is known to promote survival and the differentiation of many different cell types, exhibited a trend toward a greater improvement in SC numbers, including early angiogenesis and axonal in-growth, over ECM gel. The mechanism(s) underlying the superior effectiveness of Matrigel-suspended implants is not clear. Additional biologically active components in Matrigel, such as the growth factors EGF, bFGF, NGF, PDGF, IGF-1, and TGF-B (Davis and Stroobant, 1990; Iannotti et al., 2003), could have further enhanced the angiogenic, axon growth, and cell survival promoting proprieties of laminin and collagen. NGF plays crucial roles in both axon growth and angiogenesis (Lykissas et al., 2007; Nico et al., 2008). NGF stimulates axon growth by mediating the polymerization and accumulation of F-actin in growth cones and axon shafts (Lykissas et al., 2007), and can promote angiogenesis though its proliferation effects on endothelial cells, acting in concert with FGF and TGF to support tissue vascularization (Ohta et al., 1991) under physiological and pathological conditions. NGF can also promote cell survival through ERK and Akt activation, and downstream anti-apoptotic signaling (Perron and Bixby, 1999; Zhou et al., 2004). These different growth factors within Matrigel would be bound by the ECM and slowly released to the implanted cells and in-growing axons or other cellular components of the revascularization machinery, providing sustained effects on these processes post implantation.
In contrast, methylcellulose had less-promising results compared to the medium or laminin:collagen matrices. The poor capacity of methylcellulose to act as a supportive substrate for cell suspension grafts could be explained by inferior cell adherence and/or its inability to provide an adequate substrate for angiogenesis and axon growth. Although prior reports have indicated that methylcellulose, when used at a final concentration of 2% to bridge the injured PNS, is supportive of axon regeneration and improves nerve conductance compared to other matrices, such as laminin or collagen (Wells et al., 1997), the current study shows that methylcellulose was significantly worse than the standard practice of cell suspension in medium when employed at a slightly higher concentration of 5%; for the purpose of enhancing the mechanical strength of the in-situ gelling matrix. This increased concentration and viscosity may have restricted cell migration, the diffusion of growth factors, and axon penetrance (Labrador et al., 1995). This work demonstrates that, with synthetic matrices, defining their optimal formulations and viscosity is critical to whether they behave as an advantageous or disadvantageous suspension medium for cell implantation into the injured CNS.
In all implant groups, a small degree of cell proliferation (3–5% of the total GFP-SCs) was observed at the endpoint, 9 weeks post implantation through Ki-67 immunoreactivity (Gerdes et al., 1991); Ki-67 staining was largely confined to those cells located on the outer fringe of the implants. Although no significant difference in GFP-SC proliferation was noted, more acute differences in proliferation rather than an improved survival with laminin:collagen matrices may have accounted for the significant increase in GFP-SC numbers at endpoint. Future work looking at acute survival times following SC implantation within different suspension media may shed light on the relative contribution of enhanced GFP-SC survival versus proliferation to the significant increase in SC numbers with laminin:collagen compared to medium-only controls.
Correlation data suggested a strong association between the degree of SC survival and the amount of vascularization and axon penetrance of the injury-implant site. It appeared that the implantation of collagen:laminin matrices and their associated growth factors encouraged greater angiogenesis within the injury-implant site than medium, providing a means to support the survival of exogenous SCs through an improved supply of nutrients and oxygen. Conversely, methylcellulose suspended implants were significantly less vascularized and also exhibited the fewest number of SCs present within the injured cord. Increased axonal growth into collagen:laminin suspended implants could also have increased SC numbers through the release of known mitogenic factors for SCs; SCs do proliferate upon their exposure to axons (Wood and Bunge, 1975). Although we were unable to detect a difference in SC proliferation among implant cohorts, changes in proliferation may have been detectable at a much earlier time post implantation when axons first grew into the implants. A temporal investigation of SC numbers and their proliferation rate would identify whether such a mechanism may have played a role in the enhancement of SC numbers following collagen:laminin suspension.
DMEM-F12 medium suspension has been shown to be adequate for SC implantation, supporting cell survival, axon growth, and functional recovery (Firouzi et al., 2006; Pearse et al., 2004, 2005, 2007). Here, we demonstrate, in a clinically relevant contusion-injury model, that SC suspension within in-situ gelling laminin:collagen matrices enhances SC survival compared to medium and further improves their capacity for axon growth support, injured cord revascularization, as well as functional recovery. With cellular therapies progressing toward clinical trials for a variety of CNS diseases and trauma, as well as the known issues of obtaining adequate cell survival within the injured CNS environment (Parr et al., 2008; Pearse and Barakat, 2006; Pearse et al., 2007), these studies highlight the importance of identifying clinically applicable, in-situ gelling matrices for achieving greater survival and effectiveness of cellular implants.
Acknowledgments
We thank Raisa Puzis and David Barakat for performing immunohistochemistry; Beata Frydel and Bridgette Shaw for aid with image analysis; Yelena Pressman for her cell-culture work; Neil Masters for tissue embedding and sectioning, and slide preparation; Rosa Abril, Denise Koivisto, Monica Stagg, Maritza Garcia, Ileana Oropesa, and Andres Maldonado for help with animal care; the Viral Vector Core of The Miami Project for supplying lentiviral vectors encoding EGFP; and Alexander Marcillo, Paulo Diaz, and Michael Lynch for inducing contusion injuries.
This work was supported by: NIH NINDS Grant Number R01NS056281; The Miami Project to Cure Paralysis; The Buoniconti Fund; and The Fa Bene, Schumann, Craig H. Neilsen, and Bryon Riesch Paralysis Foundations.
Author Disclosure Statement
No competing financial interests exist.
References
- Armstrong S.J. Wiberg M. Terenghi G. Kingham P.J. ECM molecules mediate both Schwann-cell proliferation and activation to enhance neurite outgrowth. Tissue Eng. 2007;12:2863–2870. [Abstract] [Google Scholar]
- Bao F. Liu D. Peroxynitrite generated in the rat spinal cord induces neuron death and neurological deficits. Neuroscience. 2002;115:839–849. [Abstract] [Google Scholar]
- Barakat D.J. Gaglani S.M. Neravetla S.R. Sanchez A.R. Andrade C.M. Pressman Y. Bunge M.B. Pearse D.D. Survival, integration and axon growth support of glia transplanted into the chronically contused spinal cord. Cell Transplant. 2005;14:225–240. [Abstract] [Google Scholar]
- Brundin P. Karlsson J. Emgard M. Schierle G.S. Hansson O. Petersen A. Castilho R.F. Improving the survival of grafted dopaminergic neurons: A review over current approaches. Cell Transplant. 2000;9:179–195. [Abstract] [Google Scholar]
- Bunge M.B. Pearse D.D. Transplantation strategies to promote repair of the injured spinal cord. J. Rehabil. 2003;40:55–62. [Abstract] [Google Scholar]
- Chen Z.L. Strickland S. Laminin gamma1 is critical for Schwann cell differentiation, axon myelination, and regeneration in the peripheral nerve. J. Cell. Biol. 2003;163:889–899. [Europe PMC free article] [Abstract] [Google Scholar]
- Chernousov M.A. Yu W.M. Chen Z.L. Carey D.J. Strickland S. Regulation of Schwann cell function by the extracellular matrix. Glia. 2008;56:1498–1507. [Abstract] [Google Scholar]
- Cornbrooks C.J. Carey D.J. McDonald J.A. Timpl R. Bunge R.P. In vivo and in vitro observations on laminin production by Schwann cells. Proc. Natl. Acad. Sci. 1983;80:3850–3854. [Europe PMC free article] [Abstract] [Google Scholar]
- Davis J.B. Stroobant P. Platelet-derived growth factors and fibroblast growth factors are mitogens for rat Schwann cells. J. Cell Biol. 1990;110:1353–1360. [Europe PMC free article] [Abstract] [Google Scholar]
- Feng S.Q. Kong X.H. Guo S.F. Wang P. Li L. Zhong J.H. Zhou X.F. Treatment of spinal cord injury with co-grafts of genetically modified Schwann cells and fetal spinal cord cell suspension in the rat. Neurotox. Res. 2005;7:169–177. [Abstract] [Google Scholar]
- Firouzi M. Moshayedi P. Saberi H. Mobasheri H. Abolhassani F. Jahanzad I. Raza M. Transplantation of Schwann cells to subarachnoid space induces repair in contused rat spinal cord. Neurosci. Lett. 2006;402:66–70. [Abstract] [Google Scholar]
- Follenzi A. Ailles L.E. Bakovic S. Geuna M. Naldini L. Gene transfer by lentiviral vectors is limited by nuclear translocation and rescued by HIV-1 pol sequences. Nature Genetics. 2000;25:217–222. [Abstract] [Google Scholar]
- Fouad K. Schnell L. Bunge M.B. Schwab M.E. Liebscher T. Pearse D.D. Combining Schwann cell bridges and olfactory-ensheathing glia grafts with chondroitinase promotes locomotor recovery after complete transection of the spinal cord. J. Neurosci. 2005;25:1169–1178. [Europe PMC free article] [Abstract] [Google Scholar]
- Franssen E.H. de Bree F.M. Verhaagen J. Olfactory ensheathing glia: Their contribution to primary olfactory nervous system regeneration and their regenerative potential following transplantation into the injured spinal cord. Brain Res. Rev. 2007;56:236–258. [Abstract] [Google Scholar]
- Frisch S.M. Ruoslahti E. Integrins and anoikis. Curr. Opin. Cell Biol. 1997;9:701–706. [Abstract] [Google Scholar]
- Frisch S.M. Screaton R.A. Anoikis mechanisms. Curr. Opin. Cell Biol. 2001;13:555–562. [Abstract] [Google Scholar]
- Gerdes J. Li L. Schliiter C. Duchrow M. Wohlenberg C. Gerlach C. Stahmer I. Kloth S. Brandi E. Flad H.D. Immunobiochemical and molecular biologic characterization of the cell proliferation-associated nuclear antigen that is defined by monoclonal antibody Ki-67. Am. J. Pathol. 1991;138:867–873. [Europe PMC free article] [Abstract] [Google Scholar]
- Gess B. Halfter H. Kleffner I. Monje P. Athauda G. Wood P.M. Young P. Wanner I.B. Inhibition of N-cadherin and beta-catenin function reduces axon-induced Schwann cell proliferation. J. Neurosci. Res. 2008;86:797–812. [Abstract] [Google Scholar]
- Gruner J.A. A monitored contusion model of spinal cord injury in the rat. J. Neurotrauma. 1992;9:123–126. [Abstract] [Google Scholar]
- Hall E.D. Braughler J.M. Role of lipid peroxidation in post-traumatic spinal cord degeneration: A review. Cent. Nerv. Syst. Trauma. 1986;3:281–294. [Abstract] [Google Scholar]
- Han Q. Sun W. Lin H. Zhao W. Gao Y. Zhao Y. Chen B. Xiao Z. Hu W. Li Y. Yang B. Dai J. Linear ordered collagen scaffolds loaded with collagen-binding brain-derived neurotrophic factor improve the recovery of spinal cord injury in rats. Tissue Eng. Part A. 2009;15:2927–2935. [Abstract] [Google Scholar]
- Hill C.E. Hurtado A. Blits B. Bahr B.A. Wood P.M. Bartlett Bunge M. Oudega M. Early necrosis and apoptosis of Schwann cells transplanted into the injured rat spinal cord. Eur. J. Neurosci. 2007;26:1433–1445. [Abstract] [Google Scholar]
- Iannotti C. Li H. Yan P. Lu X. Wirthlin L. Xu X.M. Glial cell line-derived neurotrophic factor-enriched bridging transplants promote propriospinal axonal regeneration and enhance myelination after spinal cord injury. Exp. Neurol. 2003;183:379–393. [Abstract] [Google Scholar]
- Johnson E.O. Zoubos A.B. Soucacos P.N. Regeneration and repair of peripheral nerves. Injury. 2005;36:24–29. [Abstract] [Google Scholar]
- Kassar-Duchossoy L. Duchossoy Y. Rhrich-Haddout F. Horvat J.C. Reinnervation of a denervated skeletal muscle by spinal axons regenerating through a collagen channel directly implanted into the rat spinal cord. Brain Res. 2001;908:25–34. [Abstract] [Google Scholar]
- Kim J.E. Kim S.J. Lee B.H. Park R.W. Kim K.S. Kim I.S. Identification of motifs for cell adhesion within the repeated domains of transforming growth factor-beta-induced gene, betaig-h3. J. Boil. Chem. 2000;275:30907–30915. [Abstract] [Google Scholar]
- Labrador R.O. Buti M. Navarro X. Peripheral nerve repair: Role of agarose matrix density on functional recovery. Neuroreport. 1995;6:2022–2026. [Abstract] [Google Scholar]
- Louro J. Pearse D.D. Stem and progenitor cell therapies: Recent progress for spinal cord injury repair. Neurol. Res. 2008;30:5–16. [Abstract] [Google Scholar]
- Lykissas M.G. Batistatou A.K. Charalabopoulos K.A. Beris A.E. The role of neurotrophins in axonal growth, guidance, and regeneration. Curr. Neurovasc. Res. 2007;4:143–151. [Abstract] [Google Scholar]
- Masuda-Nakagawa L.M. Muller K.J. Nicholls J.G. Accumulation of laminin and microglial cells at sites of injury and regeneration in the central nervous system of the leech. Proc. Biol. Sci. 1990;241:201–206. [Abstract] [Google Scholar]
- McKerracher L. Chamoux M. Arregui C.O. Role of laminin and integrin interactions in growth cone guidance. Mol. Neurobiol. 1996;12:95–116. [Abstract] [Google Scholar]
- Meijs M.F. Timmers L. Pearse D.D. Tresco P.A. Bates M.L. Joosten E.A. Bunge M.B. Oudega M. Basic fibroblast growth factor promotes neuronal survival but not behavioral recovery in the transected and Schwann cell implanted rat thoracic spinal cord. J. Neurotrauma. 2004;21:1415–1430. [Abstract] [Google Scholar]
- Morrissey T.K. Kleitman N. Bunge R.P. Isolation and functional characterization of Schwann cells derived from adult peripheral nerve. J. Neurosci. 1991;11:2433–2442. [Europe PMC free article] [Abstract] [Google Scholar]
- Nandoe Tewarie R.D. Hurtado A. Levi A.D. Grotenhuis J.A. Oudega M. Bone marrow stromal cells for repair of the spinal cord: Towards clinical application. Cell Transplant. 2007;16:183. [Abstract] [Google Scholar]
- Nico B. Mangieri D. Benagiano V. Crivellato E. Ribatti D. Nerve growth factor as an angiogenic factor. Microvasc. Res. 2008;75:135–141. [Abstract] [Google Scholar]
- Ohta H. Ishiyama J. Saito H. Nishiyama N. Effects of pretreatment with basic fibroblast growth factor, epidermal growth factor and nerve growth factor on neuron survival and neovascularization of superior cervical ganglion transplanted into the third ventricle in rats. Japan. J. Pharmacol. 1991;55:255–262. [Abstract] [Google Scholar]
- Parr A.M. Kulbatski I. Zahir T. Wang X. Yue C. Keating A. Tator C.H. Transplanted adult spinal cord-derived neural stem/progenitor cells promote early functional recovery after rat spinal cord injury. Neuroscience. 2008;155:760–770. [Abstract] [Google Scholar]
- Pearse D.D. Barakat D.J. Cellular repair strategies for spinal cord injury. Expert Opin. Biol. Ther. 2006;6:639–652. [Abstract] [Google Scholar]
- Pearse D.D. Bunge M.B. Designing cell- and gene-based regeneration strategies to repair the injured spinal cord. J. Neurotrauma. 2006;23:438–452. [Abstract] [Google Scholar]
- Pearse D.D. Bushell G. Leah J.D. Jun, Fos and Krox in the thalamus after C-fiber stimulation: Coincident-input-dependent expression, expression across somatotropic boundaries, and nucleolar translocation. Neuroscience. 2001;107:143–159. [Abstract] [Google Scholar]
- Pearse D.D. Chatzipanteli K. Marcillo A.E. Bunge M.B. Dietrich W.D. Comparison of iNOS inhibition by antisense and pharmacological inhibitors after spinal cord injury. J. Neuropathol. Exp. Neurol. 2003;62:1096–1107. [Abstract] [Google Scholar]
- Pearse D.D. Lo T.P., Jr. Cho K.S. Lynch M.P. Garg M.S. Marcillo A.E. Sanchez A.R. Cruz Y. Dietrich W.D. Histopathological and behavioral characterization of a novel cervical spinal cord displacement contusion injury in the rat. J. Neurotrauma. 2005;22:680–702. [Abstract] [Google Scholar]
- Pearse D.D. Marcillo A.E. Oudega M. Lynch M.P. Wood P.M. Bunge M.B. Transplantation of Schwann cells and olfactory ensheathing glia after spinal cord injury: Does pretreatment with methylprednisolone and interleukin-10 enhance recovery? J Neurotrauma. 2004;21:1223–1239. [Abstract] [Google Scholar]
- Pearse D.D. Sanchez A.R. Pereira F.C. Andrade C.M. Puzis R. Pressman Y. Golden K. Kitay B.M. Blits B. Wood P.M. Bunge M.B. Transplantation of Schwann cells and/or olfactory ensheathing glia into the contused spinal cord: Survival, migration, axon association, and functional recovery. Glia. 2007;55:976–1000. [Abstract] [Google Scholar]
- Perron J.C. Bixby J.L. Distinct neurite outgrowth signaling pathways converge on ERK activation. Mol. Cell. Neurosci. 1999;13:362–378. [Abstract] [Google Scholar]
- Pierucci A. de Duek E.A. de Oliveira A.L. Peripheral nerve regeneration through biodegradable conduits prepared using solvent evaporation. Tissue Eng. Part A. 2008;14:595–606. [Abstract] [Google Scholar]
- Podratz J.L. Rodriguez E. Windebank A.J. Role of the extracellular matrix in myelination of peripheral nerve. Glia. 2001;35:35–40. [Abstract] [Google Scholar]
- Profyris C. Cheema S.S. Zang D. Azari M.F. Boyle K. Petratos S. Degenerative and regenerative mechanisms governing spinal cord injury. Neurobiol. Dis. 2004;15:415–436. [Abstract] [Google Scholar]
- Properzi F. Lin R. Kwok J. Naidu M. van Kuppevelt T.H. Ten Dam G.B. Camargo L.M. Raha-Chowdhury R. Furukawa Y. Mikami T. Sugahara K. Fawcett J.W. Heparan sulphate proteoglycans in glia and in the normal and injured CNS: Expression of sulphotransferases and changes in sulphation. Eur. J. Neurosci. 2008;27:593–604. [Abstract] [Google Scholar]
- Relvas J.B. Setzu A. Baron W. Buttery P.C. LaFlamme S.E. Franklin R.J. French-Constant C. Expression of dominant-negative and chimeric subunits reveals an essential role for beta1 integrin during myelination. Curr. Biol. 2001;10(11):1039–1043. [Abstract] [Google Scholar]
- Schaal S.M. Kitay B.M. Cho K.S. Lo T.P., Jr. Barakat D.J. Marcillo A.E. Sanchez A.R. Andrade C.M. Pearse D.D. Schwann cell transplantation improves reticulospinal axon growth and forelimb strength after severe cervical spinal cord contusion. Cell Transplant. 2007;16:207–228. [Abstract] [Google Scholar]
- Scholzen T. Gerdes J. The Ki-67 protein: From the known and the unknown. J. Cell Physiol. 2000;182:311–322. [Abstract] [Google Scholar]
- Serini G. Valdembri D. Bussolino F. Integrins and angiogenesis: A sticky business. Exp. Cell Res. 2006;312:651–658. [Abstract] [Google Scholar]
- Stabenfeldt S.E. García A.J. LaPlaca M.C. Thermoreversible laminin-functionalized hydrogel for neural tissue engineering. J. Biomed. Mater. Res. A. 2006;77:718–725. [Abstract] [Google Scholar]
- Stupack D.G. Integrins as a distinct subtype of dependence receptors. Cell Death Differ. 2005;12:1021–1030. [Abstract] [Google Scholar]
- Sugrue S.P. Hay E.D. The identification of extracellular matrix (ECM) binding sites on the basal surface of embryonic corneal epithelium and the effect of ECM binding on epithelial collagen production. J. Cell Biol. 1986;102:1907–1916. [Europe PMC free article] [Abstract] [Google Scholar]
- Tabesh H. Amoabediny G. Nik N.S. Heydari M. Yosefifard M. Siadat S.O. Mottaghy K. The role of biodegradable engineered scaffolds seeded with Schwann cells for spinal cord regeneration. Neurochem. Int. 2009;54:73–83. [Abstract] [Google Scholar]
- Takami T. Oudega M. Bates M.L. Wood P.M. Kleitman N. Bunge M.B. Schwann cell but not olfactory ensheathing glia transplants improve hind limb locomotor performance in the moderately contused adult rat thoracic spinal cord. J. Neurosci. 2002;22:6670–6681. [Europe PMC free article] [Abstract] [Google Scholar]
- Tate M.C. Shear D.A. Hoffman S.W. Stein D.G. LaPlaca M.C. Biocompatibility of methylcellulose-based constructs designed for intracerebral gelation following experimental traumatic brain injury. Biomaterials. 2001;22:1113–1123. [Abstract] [Google Scholar]
- Wells M.R. Kraus K. Batter D.K. Blunt D.G. Weremowitz J. Lynch S.E. Antoniades H.N. Hansson H.A. Gel matrix vehicles for growth factor application in nerve gap injuries repaired with tubes: A comparison of biomatrix, collagen, and methylcellulose. Exp. Neurol. 1997;146:395–402. [Abstract] [Google Scholar]
- Wood P.M. Bunge R.P. Evidence that sensory axons are mitogenic for Schwann cells. Nature. 1975;256:662–664. [Abstract] [Google Scholar]
- Xu X.M. Chen A. Guenard V. Kleitman N. Bunge M.B. Bridging Schwann cell transplants promote axonal regeneration from both the rostral and caudal stumps of transected adult rat spinal cord. J. Neurocytol. 1997;26:1–16. [Abstract] [Google Scholar]
- Yoshii S. Ito S. Shima M. Taniguchi A. Akagi M. Functional restoration of rabbit spinal cord using collagen-filament scaffold. J. Tissue Eng. Regen. Med. 2009;3:19–25. [Abstract] [Google Scholar]
- Zhou F.Q. Zhou J. Dedhar S. Wu Y.H. Snider W.D. NGF-induced axon growth is mediated by localized inactivation of GSK-3beta and functions of the microtubule plus end binding protein APC. Neuron. 2004;42:897–912. [Abstract] [Google Scholar]
Articles from Journal of Neurotrauma are provided here courtesy of Mary Ann Liebert, Inc.
Full text links
Read article at publisher's site: https://doi.org/10.1089/neu.2008.0809
Read article for free, from open access legal sources, via Unpaywall:
https://europepmc.org/articles/pmc2943946?pdf=render
Citations & impact
Impact metrics
Citations of article over time
Article citations
Schwann Cell-Derived Exosomal Vesicles: A Promising Therapy for the Injured Spinal Cord.
Int J Mol Sci, 24(24):17317, 10 Dec 2023
Cited by: 3 articles | PMID: 38139147 | PMCID: PMC10743801
Review Free full text in Europe PMC
Injectable hydrogels in central nervous system: Unique and novel platforms for promoting extracellular matrix remodeling and tissue engineering.
Mater Today Bio, 20:100614, 22 Mar 2023
Cited by: 19 articles | PMID: 37008830 | PMCID: PMC10050787
Review Free full text in Europe PMC
Biomaterial-Based Schwann Cell Transplantation and Schwann Cell-Derived Biomaterials for Nerve Regeneration.
Front Cell Neurosci, 16:926222, 28 Jun 2022
Cited by: 14 articles | PMID: 35836742 | PMCID: PMC9273721
Review Free full text in Europe PMC
Biomaterial and Therapeutic Approaches for the Manipulation of Macrophage Phenotype in Peripheral and Central Nerve Repair.
Pharmaceutics, 13(12):2161, 15 Dec 2021
Cited by: 11 articles | PMID: 34959446 | PMCID: PMC8706646
Review Free full text in Europe PMC
Recombinant Adenoviruses for Delivery of Therapeutics Following Spinal Cord Injury.
Front Pharmacol, 12:777628, 10 Jan 2022
Cited by: 3 articles | PMID: 35082666 | PMCID: PMC8784517
Review Free full text in Europe PMC
Go to all (38) article citations
Similar Articles
To arrive at the top five similar articles we use a word-weighted algorithm to compare words from the Title and Abstract of each citation.
Early profiles of axonal growth and astroglial response after spinal cord hemisection and implantation of Schwann cell-seeded guidance channels in adult rats.
J Neurosci Res, 82(4):472-483, 01 Nov 2005
Cited by: 21 articles | PMID: 16240391
Biodegradable poly-beta-hydroxybutyrate scaffold seeded with Schwann cells to promote spinal cord repair.
Biomaterials, 29(9):1198-1206, 01 Mar 2008
Cited by: 89 articles | PMID: 18083223
Regrowth of axons into the distal spinal cord through a Schwann-cell-seeded mini-channel implanted into hemisected adult rat spinal cord.
Eur J Neurosci, 11(5):1723-1740, 01 May 1999
Cited by: 158 articles | PMID: 10215926
Schwann cells for spinal cord repair.
Braz J Med Biol Res, 38(6):825-835, 01 Jun 2005
Cited by: 31 articles | PMID: 15933775
Review
Funding
Funders who supported this work.
NINDS NIH HHS (2)
Grant ID: R01NS056281
Grant ID: R01 NS056281