Abstract
Free full text
Matrix Metalloproteinase-Induced Epithelial-Mesenchymal Transition in Breast Cancer
Abstract
Matrix metalloproteinases (MMPs) degrade and modify the extracellular matrix (ECM) as well as cell-ECM and cell-cell contacts, facilitating detachment of epithelial cells from the surrounding tissue. MMPs play key functions in embryonic development and mammary gland branching morphogenesis, but they are also upregulated in breast cancer, where they stimulate tumorigenesis, cancer cell invasion and metastasis. MMPs have been investigated as potential targets for cancer therapy, but clinical trials using broad-spectrum MMP inhibitors yielded disappointing results, due in part to lack of specificity toward individual MMPs and specific stages of tumor development. Epithelial-mesenchymal transition (EMT) is a developmental process in which epithelial cells take on the characteristics of invasive mesenchymal cells, and activation of EMT has been implicated in tumor progression. Recent findings have implicated MMPs as promoters and mediators of developmental and pathogenic EMT processes in the breast. In this review, we will summarize recent studies showing how MMPs activate EMT in mammary gland development and in breast cancer, and how MMPs mediate breast cancer cell motility, invasion, and EMT-driven breast cancer progression. We also suggest approaches to inhibit these MMP-mediated malignant processes for therapeutic benefit.
Matrix Metalloproteinases: Overview
There are 23 human MMPs (Degradome database; http://degradome.uniovi.es) [1], including 17 soluble, secreted enzymes and 6 membrane-associated enzymes (Fig. 1); they differ from each other in their structural domain architecture, in their substrate specificity, and in their temporal and tissue specific expression patterns. MMPs were originally named for their preferred substrates within the extracellular matrix (ECM): collagen-cleaving MMPs (MMP-1, -8, and -13) were designated collagenases, gelatin (denatured collagen)-cleaving MMPs (MMP-2 and -9) were termed gelatinases, and MMPs that degraded a broad spectrum of ECM proteins were called stromelysins (MMP-3, -10, and -11) or matrilysins (MMP-7). As the MMP family grew with the discovery of additional paralogs, including the membrane-associated MMPs, of which MT1-MMP/MMP-14 is the founding member, a numbering system was adopted, and MMPs are now grouped according to their domain structure.
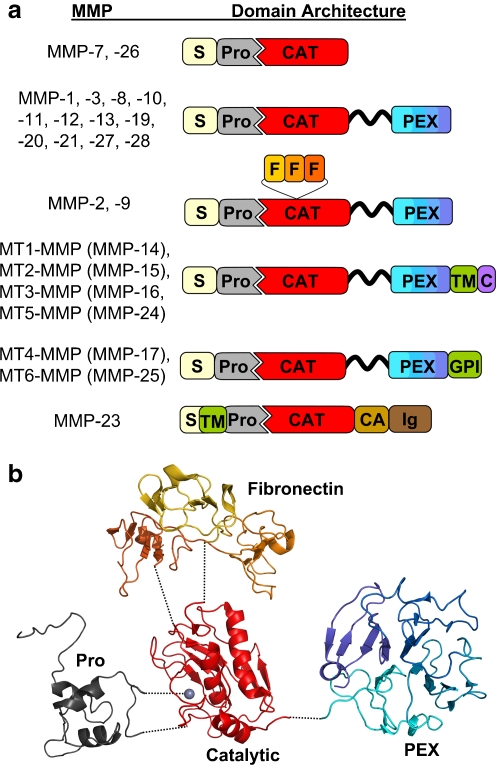
MMP domain structure and protein fold. a The various domain organizations of human MMPs are illustrated; S, signal peptide; Pro, propeptide; CAT, catalytic domain; F, fibronectin repeats; PEX, hemopexin domain; TM, transmembrane domain; GPI, glycophosphatidylinositol membrane anchor; C, cytoplasmic domain; CA, cysteine array; Ig, immunoglobulin-like domain. The flexible, variable length linker or hinge region is depicted as a wavy black ribbon. b The protein structure of the domains of a representative proMMP (proMMP-2) is shown; the individual domains, colored as in the cartoon above, have been separated for visual clarity. Dotted lines indicate the coordination of prodomain cysteine to the catalytic zinc (gray sphere), as well as the points of covalent attachment between the catalytic domain and the prodomain, fibronectin repeats, and PEX domain. Figure was generated with Pymol [155], using coordinates from Protein Databank entry 1GXD [156].
MMPs are modular enzymes (Fig. 1a). The core functional domain of every MMP is the catalytic domain, a compact globular domain of 160–170 amino acids featuring a highly conserved HExxHxxGxxH zinc binding motif, responsible for chelating the catalytically essential zinc ion at the enzyme active site [2]. The catalytic zinc and substrate binding cleft of the catalytic domain comprise the MMP region targeted for binding and inhibition by the endogenous tissue inhibitors of metalloproteinases (TIMPs) [3], and also by the majority of small-molecule, synthetic pharmaceutical inhibitors of MMPs [4]. MMPs are produced as latent proenzymes, in which an N-terminal prodomain of ~80 amino acids blocks catalytic activity by physically blocking the active site, through coordination of a conserved cysteine residue within a PRCGxPD motif (“the cysteine switch”) to the catalytic zinc [2, 5]. Upon stepwise interaction with and cleavage by one or more activating proteases [5, 6], the cleaved MMP prodomain dissociates from the catalytic domain, releasing the active enzyme.
Most MMPs also possess additional accessory domains that act to modulate catalytic activity, substrate recognition, and cellular localization [3, 7]; some accessory domains may also confer non-catalytic functions of potential significance for understanding MMP roles in EMT and tumor progression. The gelatinases MMP-2 and -9 are assisted in substrate binding of gelatin, collagens, and laminin by three fibronectin repeats inserted into the catalytic domain [8]. All human MMPs with the exception of MMP-7, -23, and -26A possess a C-terminal hemopexin (PEX) domain, a four-bladed propeller structure that is connected to the catalytic domain by a flexible linker [9, 10]. PEX domains have been shown to mediate the binding and unwinding of collagen triple helices by collagenases, facilitating cleavage by the MMP catalytic domain [11–14], as well as the recognition of other substrates including gelatin binding by MMP-9 [15], fibrinogen binding by MMP-2 [16], and targeting of several chemokines by MMP-2 [17–19]. Beyond substrate recognition, PEX domains can mediate interactions with tissue inhibitors of metalloproteinases (TIMPs), with distinctly different results for different MMP/TIMP pairs: proMMP-2 is targeted for MT1-MMP-mediated activation by TIMP-2 [3, 5], while proMMP-9, by binding to TIMP-1, is protected from activation [20–22].
In mammary epithelial cells, specific interactions between PEX domains and integrins or other cell surface receptors have been found to facilitate MMP activation, to localize soluble MMPs to sites of pericellular proteolysis, or to regulate MMP endocytosis and turnover [10]. MMP-9 docking to the hyaluronan receptor CD44 mediates proteolytic activation of TGF-β and promotion of tumor invasion and angiogenesis in a murine mammary carcinoma model [23]. Association of the MT1-MMP PEX domain with CD44H leads to localization of MT1-MMP at the leading edge of migrating cells [24] and facilitates cell migration [25]. Interaction between MT1-MMP and CD44 also stimulated epithelial cell self-sorting in an engineered model of mammary ductal morphogenesis; this function did not appear to depend upon MMP catalytic activity [26].
MMPs Stimulate Breast Cancer Progression
While a finely tuned array of MMPs is instrumental in orchestrating tissue development and homeostasis, the misregulation of MMPs is widespread in many pathological settings and especially in cancer, where MMP overexpression contributes to tumorigenesis and tumor progression through multiple mechanisms. MMP proteolysis serves a path-clearing role in facilitating the movement of cells or groups of cells through ECM [27, 28]; in this process, cleavage of some ECM components unmasks cryptic sites, generating fragments with new biological activities modulating migration, growth, or angiogenesis [27, 28]. MMPs also cleave cell-ECM adhesion proteins and cell-cell junction proteins, releasing individual epithelial cells from epithelial sheets, initiating outside-in signaling pathways that lead to widespread changes in gene transcription patterns, or generating soluble ectodomain fragments with novel activities. MMP-1 cleaves and activates the protease activated receptor-1 (PAR-1), leading to increased migration and invasion of breast cancer cells [29]. Targeting of E-cadherin by MMP-3 or MMP-7 generates a bioactive fragment that promotes invasion [30], and contributes to a cascade of molecular alterations leading to EMT in mammary epithelial cells [30, 31]. MT1-MMP processing of αv integrin enhances breast cancer cell migration [32, 33], and MMP shedding of the ectodomain of P-cadherin facilitates breast cancer cell invasion [34]. MMPs can also promote breast tumor progression by targeting soluble molecules. Examples include protease activation cascades (activation of MMP-9 by MMP-3 [22], activation of MMP-2 by MT1-MMP [35]), the activation of latent TGF-β by MMP-2 and MMP-9 [23], and the N-terminal truncation of interleukin-8 (IL-8) by MMP-9, increasing its neutrophil-activating potential by an order of magnitude [36].
Transgenic or knockout mouse models have been used to establish specific effects of individual MMPs on mammary tumor development (reviewed in [37]). MMP-3 overexpression driven by the whey acidic protein (WAP) promoter, most active in mammary gland epithelial cells from mid-pregnancy and during lactation, led to widespread premalignant alterations and spontaneous tumor formation [38]. Similarly, overexpression of MMP-7 under control of the mouse mammary tumor virus (MMTV) promoter, which is active during puberty and greatly enhanced during pregnancy, resulted in spontaneous formation of premalignant mammary hyperplasias, and accelerated tumor formation in bitransgenic MMTV-MMP-7/neu mice [39]. MMTV-driven overexpression of MT1-MMP also led to premalignant mammary gland abnormalities and spontaneous adenocarcinoma [40]. In studies with MMP-11 knockout mice subjected to 7,12-dimethylbenzanthracene (DMBA)-induced carcinogenesis, MMP-11 null mice developed fewer tumors [41]; MMP-11 null mice also developed fewer spontaneous tumors in the mammary gland cancer prone MMTV-ras model [42]. One clear conclusion from these studies is that some MMPs can act as tumor promoters in mammary carcinogenesis, impacting neoplastic risk from the very earliest stages of premalignant change [38, 43].
MMPs can also modulate later stages of cancer progression in genetic models of breast cancer. For example, knockout of MMP-9 in the MMTV-polyoma virus middle T-antigen (PyMT or PyVT) multistage mammary tumorigenesis model resulted in an 80% reduction in lung metastatic burden, indicating the importance of MMP-9 in metastasis and angiogenesis in this model [44]. In another study employing the MMTV-PyMT tumorigenesis model, MT1-MMP null mammary glands transplanted into syngeneic mice developed tumors with a markedly reduced capacity to metastasize to the lungs, compared with an MT1-MMP sufficient control group, demonstrating a role for tumor MT1-MMP in the metastatic process [45]. In a study investigating the roles of MMPs in recruitment of stromal bone-resorbing osteoclasts to breast-to-bone metastases, mammary tumor cells implanted into the bones of MMP-7 null mice formed smaller, slower growing tumors with recruitment of fewer osteoclasts and less osteolysis, implicating MMP-7 in this aspect of metastatic progression [46].
Of course, MMPs do not act as universal tumor promoters under all circumstances, and the effects observed can also vary depending upon the model and upon the genetic background of the mice [43, 44]. In contrast with the protumorigenic effects of MMP-3 in the WAP promoter model [38], MMTV-driven MMP-3 expression in a different strain of mice did not lead to spontaneous tumorigenesis, and in a DMBA chemical carcinogenesis protocol, MMTV-MMP-e mice were reported to have fewer mammary gland tumors and more apoptotic cells [43, 47]. In contrast with the tumor promoting effect of MT1-MMP in mice with mammary-directed overexpression of this protease [40], MT1-MMP had a growth suppressing effect in the MMTV-PyMT genetically induced model when tumorigenesis in MT1-MMP null versus MT1-MMP sufficient mammary glands were compared [45]. For MMP-9 promotion of breast-to-lung metastasis as well, the genetic background of the mice was a determining factor, as C57BL/6 mice showed MMP-9 dependent promotion of metastatic growth, whereas no significant differences were observed between wt and MMP-9 null mice of the FVB/N background [44]. These observations underscore the complexity of the process of tumor development, in which MMPs must interact with many other variables; it has been suggested that as in mice, genetic modifiers present in human patient populations may distinguish subgroups likely to benefit from therapeutic intervention in MMP-mediated processes [44].
MMPs and Physiological EMT
EMT is a process integral to the formation of many tissues and organs during development [48–51]. Activation of developmental EMT has been found to follow a defined sequence of events: morphogenesis of the epithelial tissue and specification of the cells that will undergo EMT, disruption or degradation of the basement membrane, breakdown of the epithelial tissue structure followed by ingression of the separated cells, and differentiation to the motile mesenchymal phenotype [49]. While MMPs have long been suspected to play roles in many different EMT-related tissue morphogenesis and cell migration processes, direct evidence of MMP involvement has been best characterized for neural crest delamination, endocardial cushion invasion, and mammary gland branching morphogenesis. EMT of the neural crest during embryogenesis releases mesenchymal cells that migrate through the body, giving rise to a wide variety of tissue types, including glial and neuronal cells, adrenal glandular tissues, melanocytes, and skeletal and connective tissues [49, 52] (Fig. 2a). MMP-2 becomes activated in the neural cells as they are undergoing EMT, but inactivated as the cells begin to disperse [53–56]; blocking MMP-2 inhibits EMT without affecting the migration of the detached neural crest cells [53]. EMT of embryonic endocardial cells into the endocardial cushion creates precursors of the valvular and septal structures (Fig. 2b) [57], and also is dependent upon expression of MMP-2, as treatment with MMP inhibitors blocks mesenchyme formation [58, 59]. Studies using endocardial cushion explants grown on collagen gels revealed that MMP-2-dependent EMT involves degradation of collagen-IV [58], and requires specific association of MMP-2 with integrin αvβ3 [60].
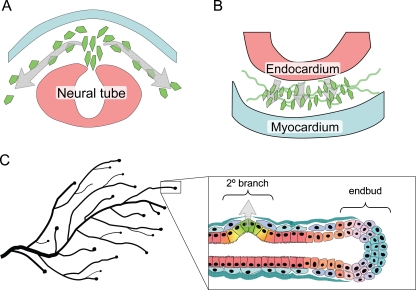
MMP-induced EMT in development. a. Neural crest delamination is facilitated by expression of MMP-2 in the cells undergoing EMT. b. EMT of endocardial cushions during early stages of heart development depends upon expression of MMP-2 in the endocardial cells. c. Secondary (2°) branching of the mammary ductal tree involves breakdown of epithelial structure, acquisition of invasive characteristics, and degradation of the basement membrane, processes shown to be dependent upon MMP-3, which is produced locally in response to morphogenic signals by the surrounding stromal cells.
Unlike many other tissues, the majority of mammary gland development occurs postnatally. During puberty, the rudimentary mammary gland grows into the fat pad through ductal extension and branching morphogenesis [61–63]. Extension of the ducts into the fat pad occurs at the endbuds, invasive structures that express high levels of EMT-associated transcription factors, including Snail and Twist [64] as well as MMP-2 and MT1-MMP [65]. Mammary branching morphogenesis occurs by two distinct mechanisms: primary branching through endbud bifurcation, and secondary branching, a process strikingly similar to developmental EMT, in which differentiated, ductal epithelium dedifferentiates, detaches from the adjacent epithelial cells, penetrates the basement membrane, and invades into the surrounding tissue (Fig. 2c). MMP-3 is a key mediator of secondary branch formation, as transgenic mice lacking MMP-3 expression have significantly reduced secondary branching, while the WAP-MMP-3 mice have increased secondary branching and ductal complexity [65, 66]. Upregulation of MMP-3 has also been implicated in the increased side-branching observed in transgenic mice in which retinoic acid signaling pathways are inhibited [67]. The mechanism by which MMP-3 induces branching morphogenesis has been investigated in 3D culture models in which mouse mammary epithelial cell clusters are grown in collagen I gels. These studies have shown that epimorphin, a stromal cell-produced morphogen, induces expression of MMP-3, and that this is both necessary and sufficient for activation of the branching process [68–71]. Activation of the fibroblast growth receptor signaling pathway, which has also been implicated in mammary branching morphogenesis, also induced MMP-3 expression and branch initiation in mammary epithelial cells grown in 3D collagen [72].
MMPs and EMT in Breast Cancer
MMPs have been associated with EMT in cancer progression through three distinct mechanisms: (a) elevated levels of MMPs in the tumor microenvironment can directly induce EMT in epithelial cells, (b) cancer cells that undergo EMT can produce more MMPs, facilitating cell invasion and metastasis, and (c) EMT can generate activated stromal-like cells that drive cancer progression via further MMP production. The most dramatic of these is MMP-dependent activation of the EMT program (Fig. 3a), seen in a variety of epithelial cell types, including kidney [73–76], ovary [77], lens [78], lung [79], and prostate [80], although MMP-induced EMT has been best characterized in mammary epithelial cells. Tumors that developed in the WAP-MMP-3 mice showed mesenchymal characteristics [38, 81, 82], and dissection of this process revealed that exposure of cultured mouse mammary epithelial cells to MMP-3 directly activates EMT [31, 83]. MMP-3 mediates these effects by stimulating increased expression of Rac1b [84], a constitutively activated splice variant of Rac1 found in breast and colorectal cancer cells [85–89], which in turn triggers EMT by increasing levels of cellular reactive oxygen species [84, 90]. While the process by which MMP-3 initiates these effects has not been completely defined, MMP-3 has been shown to cleave E-cadherin, promoting dissolution of epithelial cells and releasing a bioactive fragment of E-cadherin that induces cell motility [30, 31]. It is likely that many studies in which MMPs have been seen to stimulate cancer cell motility and invasion, although not directly investigating these phenomena in the context of EMT, have in fact been observing the cellular consequences of an incomplete activation of the EMT program. Unlike developmental EMT, where MMPs are a component of an organized morphogenic program, the chaotic and MMP-enriched tumor microenvironment induces an uncoordinated and incomplete EMT. As a consequence, these EMT-activated cells may acquire significant tumor-promoting abilities even as they retain many of their original characteristics, making it difficult to distinguish them from the original tumor mass from which they are derived.
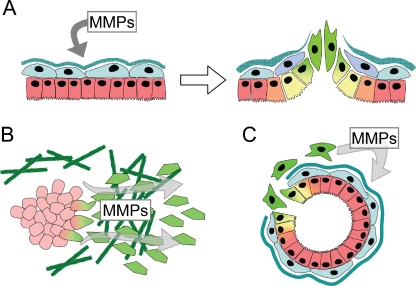
MMPs facilitate EMT-associated tumor progression. a. Exposure of epithelial cells to MMPs can directly induce EMT. b. Increased expression of MMPs in cells which have undergone EMT facilitates cancer cell invasion. c. EMT can produce nonmalignant stromal cells which drive tumor initiation and progression through production of MMPs.
Breast cancer cells which have undergone EMT also show increased expression of MMPs, facilitating their invasive, metastatic characteristics (Fig. 3b; as this topic was comprehensively reviewed in 2005 [91], only highlights and more recent studies will be covered here). Early investigations revealed that breast cancer cell lines expressing mesenchymal markers often expressed MMPs, and that suppression of these MMPs blocked their invasive and migratory characteristics [92–94]. Subsequent investigations have identified MMP upregulation associated with a variety of EMT processes, although the specific MMPs induced seem to depend upon the nature of the EMT-inducing agent and the model system used. Transcriptional profiling studies of Ras-transformed mouse mammary epithelial cells induced to undergo EMT by treatment with TGFβ revealed MMP-2, MMP-12, and MMP-13 among the most upregulated transcripts [95, 96]. Culture of MCF10A cells at low density activated EMT-like changes associated with increased expression of MT1-MMP [97, 98], while induction of EMT in MCF10A cells by exposure to TGFβ or expression of ErbB2 stimulated expression of MMP-2 and MMP-9, respectively [99, 100]. Decreased expression of singleminded-2s in mouse mammary epithelial cells or in MCF-7 breast cancer cells activated EMT and expression of MMP-2 [101], while expression of Snail in MCF-7 cells induced an MT1-MMP and MT2-MMP-dependent invasion program [102]. Activation of EMT in NMuMg cells by treatment with hydrogen peroxide led to activation of MMP-3, MMP-10, and MMP-13 [103], while induction of EMT by the Abl tyrosine kinase in the same cell line led to MMP-3 and MMP-9 expression [104]. As breast cancer progression is a complex process, it may be unsurprising that distinct profiles of MMPs are activated in systems that model different breast cancer stages and disease subtypes.
EMT of cancer cells may produce stromal-like derivatives that, while not intrinsically malignant, act to facilitate tumor progression through production of MMPs (Fig. 3c). Myofibroblasts are principal components of the reactive stroma surrounding breast cancers, and these cells have been found to have powerful tumor-promoting characteristics [105–108]. While myofibroblasts can be produced through activation of stromal fibroblasts or circulating fibrocytes, recent studies using mouse models have shown that myofibroblasts can be derived from epithelial cells by EMT [107, 109–113]. EMT functions in human breast cancer as well: stromal-like and myofibroblast-like cells surrounding breast tumors have been found to be derived from the epithelial cancer cells [107, 114]. It is further known that mammographic density, an established risk factor for and potential precursor of breast cancer [115, 116], is associated with fibroblast accumulation [117–122]. Studies of MMP localization in human tumors have shown that stromal fibroblasts are a major contributor to the production of many MMPs [123–125], and tumor progression and poor prognosis is associated with stromal expression of MMP-1, MMP-7, and MMP-12 [126], and with fibroblast-specific production of MMP-9, MMP-11, and MT1-MMP [124, 125]. Further defining which MMPs are produced by breast cancer-associated myofibroblasts, and how these MMPs act in tumor progression, will provide insight into how EMT-driven tumor progression can best be targeted therapeutically.
Unfortunately, there have been very few studies that assess histological correlates of EMT with expression of MMPs in human breast tissues. Studies with metaplastic breast carcinoma, a relatively uncommon subtype for which ongoing EMT processes are evident, have found that stromelysin-3/MMP-11 expression in epithelial cells is a prognostic factor for disease progression—patients who expressed more MMP-11 in epithelial carcinoma cells had significantly shorter disease-free survival [127]. More recently, profiling studies of metaplastic breast carcinoma have found that altered expression of MMPs and TIMPS were found in patients with more rapid disease progression [128]. However, a direct connection between MMPs and EMT that can be assessed by histological characteristics awaits future research.
Therapeutic Targeting of MMP-promoted EMT
An obvious point for intervention in MMP-induced or mediated EMT is the catalytic inhibition of MMPs themselves. Unfortunately, clinical trials of first- and second-generation small molecule MMP-inhibiting drugs in breast cancer and other cancers proved disappointing [129]. A phase III trial of the MMP inhibitor marimastat in patients with metastatic breast cancer found no therapeutic benefit [130], while phase II trials of marimastat and rebimastat in patients with early-stage breast cancer concluded that large adjuvant trials with these agents were not feasible due to musculoskeletal toxicity and failure to achieve therapeutic plasma levels [131, 132]. Many of the problems with the MMP inhibitors tested to date appear to stem in large part from a lack of specificity; the drugs employed simply target too many enzymes. This is a critical problem, because some MMPs appear to protect against tumor progression at certain stages of breast cancer development, and inhibition of these MMPs at the wrong time can lead to increased tumor aggressiveness [27, 133–135]. For example, high levels of MMP-8 have been shown to suppress breast cancer metastasis [136], potentially by increasing tumor cell adhesion to ECM and diminishing cellular invasive potential [137]; significantly, ribozyme-mediated knockdown of MMP-8 in a nonmetastatic, high MMP-8 breast cancer cell line conferred metastatic competence [136]. Thus, pharmacological inhibition of MMP-8 along with invasion- and metastasis-promoting MMPs would be anticipated to reduce or limit the potential benefit of the therapy.
As another consequence of poor specificity, clinical trials of MMP inhibitors were plagued by the serious side effect of musculoskeletal syndrome (MSS). This dose-limiting toxicity frequently resulted in failure to achieve targeted plasma levels, and in patients withdrawing from treatment, further compromising the statistical significance of trial outcomes [138]. The specific molecular target responsible for these side effects has not been conclusively identified; early candidates included MMP-1 and the ADAM family of metalloproteases, but synthetic inhibitors developed to minimize inhibition of these targets still produced MSS symptoms [138]. Remaining candidate mediators of MSS include MT1-MMP [135], metalloproteases outside of the MMP and ADAM families [139], or nonprotease metalloproteins [138]. To minimize off-target effects, well-tolerated MMP-directed therapeutics will need to achieve selectivity for the MMP family in preference to other metalloenzymes, as well as the ability to distinguish among MMPs.
The key challenges yet to be surmounted to bring MMP inhibitors to the clinic as an approach to combat MMP-mediated EMT and resulting cancer progression are (1) identification of the individual MMP targets implicated as primary drivers of EMT-promoted malignancy at specific points in tumor progression, and (2) development of therapeutic molecules capable of targeting these cancer-driving MMPs with exquisite selectivity. An attempt has already been made to synthesize existing data from current models into a master list of MMP drug targets versus “anti-targets”, for which pharmacological intervention would be presumed counterproductive [135]. However, the data currently available are insufficient to support definitive classification of most MMPs, particularly as questions remain regarding the extent to which various animal cancer models fully and faithfully reproduce the functional diversity of individual MMPs in cancer progression in humans. In particular, MMP-3, MMP-9, and MT1-MMP have all been suggested as drug anti-targets due to reports of antitumor effects associated with these MMPs in some model systems [135]; yet, the bulk of the literature supports the view that these are among the MMPs most directly implicated in promoting EMT, motility, invasion, and metastasis in cancer models. Rather than eliminating these MMPs from the drug target lineup, it would instead be prudent to cautiously pursue them, keeping in mind that further basic research into their functions in tumor development is necessary, and that their effective targeting for therapeutic benefit will require careful definition of the patient populations most likely to benefit, with regard to disease stage, pathological characteristics, and potential genetic modifiers. As an example of stage-specific considerations of potential importance in targeting MMP-induced EMT, it has been observed that MMP-3-induced EMT of breast epithelial cells is initially reversible upon withdrawal of the MMP, but eventually becomes permanent [31, 38], suggesting that therapeutic intervention with MMP inhibitors may be most effective at early stages of breast cancer development.
In the arena of more highly selective small molecule MMP inhibitors, slow progress is being made. These synthetic compounds typically feature a zinc-chelating group such as hydroxamate derivitized with peptidic or nonpeptidic groups designed to mimic a peptide substrate; they target the MMP active site zinc and substrate binding site [4, 140, 141]. Structure-based design of selective inhibitors has been hampered by the close structural homology of active sites and overlapping substrate specificities among the MMPs, and by the elastic and flexible nature of the MMP active site, which further complicates computational drug design even when high resolution crystal structures are available [141–144]. Current approaches to small molecule MMP inhibitors include optimization of compounds based on an array of different zinc-binding groups to yield more selective inhibitors toward a variety of MMPs [4, 145], as well as the development of non-zinc-binding inhibitors that selectively target unique aspects of the MMP-13 active site [145]. A less conventional approach has pursued development of irreversible mechanism-based inhibitors, selective for gelatinases MMP-2 and MMP-9, that covalently modify the catalytic glutamate residue of the MMP active site [145, 146]. In yet another approach, several groups have attempted to exploit the selective substrate binding exosites present on MMP accessory domains to develop selective allosteric inhibitors of MMPs; while a promising concept, this approach has yet to yield highly potent and selective drug leads [7].
An alternative to small molecule MMP inhibitors is presented by macromolecular protein therapeutics. Promising candidates for development include engineered variants of the natural MMP-inhibiting TIMPs, and MMP-targeting therapeutic antibodies. TIMPs offer the advantage of an extensive contact surface ideally evolved for high affinity interaction with MMP targets. Although the four native TIMPs possess only a limited ability to differentiate between the many members of the MMP family, mutational studies have established the potential for modulating binding specificity by alteration of key residues at the MMP-TIMP interface [147–152]. A recombinant triple mutant variant of the TIMP-1N-terminal domain, optimized for selectivity to MT1-MMP, was recently found to potently block MT1-MMP collagenase activity and CD44 shedding in breast cancer and fibrosarcoma cell culture models [153]. In another approach to selective MMP inhibition, several function blocking antibodies have been reported that selectively target individual MMPs [7]. In one recent and promising example, phage display technology was used to identify an MT1-MMP-selective human monoclonal antibody that blocked the proteolytic activity of the enzyme; this protein therapeutic was found to slow tumor progression and metastasis in an orthotopic xenograft model of breast cancer [154].
Thus, the challenges are clear: while some MMPs facilitate breast cancer development and could potentially be targeted for therapeutic benefit, others are essential for basic physiological processes, interference with which can have serious negative consequences. We need methods to target specific MMPs, as well as a much better understanding of which MMPs to target and when. Furthermore, while much has been learned about how to target the catalytic activities of MMPs, recent research has revealed that their noncatalytic accessory functions must also be considered. The efforts of chemists, biologists, bioengineers, and physicians must now be combined to discover selective drugs and reagents, to create the most informative experimental models in which to dissect the roles of MMPs in EMT-driven breast cancer progression, to develop and test optimal intervention strategies, and to effect the translation of these therapies into the clinic.
Financial Support
This work is supported by grants from the National Cancer Institute (CA122086, CA128660, and CA132879 to DCR), from the James and Esther King Foundation (07KN09 to DCR; 08KN12 to ESR), from the Bankhead-Coley Foundation (09BB17 to ESR), from the Susan B. Komen foundation (FAS0703855 to DCR), and by the Mayo Clinic Breast Cancer Specialized Program of Research Excellence (SPORE) grant CA116201 (PI James Ingle MD) from the National Institutes of Health.
Open Access
This article is distributed under the terms of the Creative Commons Attribution Noncommercial License which permits any noncommercial use, distribution, and reproduction in any medium, provided the original author(s) and source are credited.
Abbreviations
EMT | epithelial-mesenchymal transition |
MMP | matrix metalloproteinase |
ECM | extracellular matrix |
TIMP | tissue inhibitor of metalloproteinase |
PEX | hemopexin |
TGF-β | transforming growth factor-β |
LRP | lipoprotein receptor-related protein |
WAP | whey acidic protein |
MMTV | mouse mammary tumor virus |
PyMT | polyoma virus middle T-antigen |
Contributor Information
Evette S. Radisky, Email: [email protected].
Derek C. Radisky, Phone: +1-904-9536372, Fax: +1-904-9530277, Email: [email protected].
References
Full text links
Read article at publisher's site: https://doi.org/10.1007/s10911-010-9177-x
Read article for free, from open access legal sources, via Unpaywall:
https://link.springer.com/content/pdf/10.1007/s10911-010-9177-x.pdf
Citations & impact
Impact metrics
Article citations
Intricate relationship between cancer stemness, metastasis, and drug resistance.
MedComm (2020), 5(10):e710, 21 Sep 2024
Cited by: 0 articles | PMID: 39309691 | PMCID: PMC11416093
Review Free full text in Europe PMC
The SEMA3F-NRP1/NRP2 axis is a key factor in the acquisition of invasive traits in in situ breast ductal carcinoma.
Breast Cancer Res, 26(1):122, 13 Aug 2024
Cited by: 0 articles | PMID: 39138514 | PMCID: PMC11320849
TSP50 facilitates breast cancer stem cell-like properties maintenance and epithelial-mesenchymal transition via PI3K p110α mediated activation of AKT signaling pathway.
J Exp Clin Cancer Res, 43(1):201, 20 Jul 2024
Cited by: 0 articles | PMID: 39030572 | PMCID: PMC11264956
Proteogenomic characterization of pancreatic neuroendocrine tumors uncovers hypoxia and immune signatures in clinically aggressive subtypes.
iScience, 27(8):110544, 20 Jul 2024
Cited by: 0 articles | PMID: 39206147 | PMCID: PMC11350455
The Effect of NF-κB Deactivation on Cancer Cell Response to ALA Mediated Photodynamic Therapy.
Asian Pac J Cancer Prev, 25(6):2051-2058, 01 Jun 2024
Cited by: 0 articles | PMID: 38918667 | PMCID: PMC11382837
Go to all (281) article citations
Data
Data behind the article
This data has been text mined from the article, or deposited into data resources.
BioStudies: supplemental material and supporting data
Protein structures in PDBe
-
(1 citation)
PDBe - 1GXDView structure
Similar Articles
To arrive at the top five similar articles we use a word-weighted algorithm to compare words from the Title and Abstract of each citation.
The pathophysiology of epithelial-mesenchymal transition induced by transforming growth factor-beta in normal and malignant mammary epithelial cells.
J Mammary Gland Biol Neoplasia, 15(2):169-190, 15 May 2010
Cited by: 154 articles | PMID: 20467795 | PMCID: PMC3721368
Review Free full text in Europe PMC
microRNAs and EMT in mammary cells and breast cancer.
J Mammary Gland Biol Neoplasia, 15(2):213-223, 25 May 2010
Cited by: 39 articles | PMID: 20499142
Review
ErbB/EGF signaling and EMT in mammary development and breast cancer.
J Mammary Gland Biol Neoplasia, 15(2):191-199, 06 Apr 2010
Cited by: 97 articles | PMID: 20369376 | PMCID: PMC2889136
Review Free full text in Europe PMC
Epithelial-mesenchymal transition in cancer: parallels between normal development and tumor progression.
J Mammary Gland Biol Neoplasia, 15(2):117-134, 19 May 2010
Cited by: 594 articles | PMID: 20490631 | PMCID: PMC2886089
Review Free full text in Europe PMC
Funding
Funders who supported this work.
NCI NIH HHS (8)
Grant ID: CA128660
Grant ID: R01 CA122086
Grant ID: R01 CA132879
Grant ID: CA122086
Grant ID: P50 CA116201
Grant ID: CA132879
Grant ID: R21 CA128660
Grant ID: CA116201