Abstract
Free full text

Cellular, Molecular, and Genetic Substrates Underlying the Impact of Nicotine on Learning
Abstract
Addiction is a chronic disorder marked by long-lasting maladaptive changes in behavior and in reward system function. However, the factors that contribute to the behavioral and biological changes that occur with addiction are complex and go beyond reward. Addiction involves changes in cognitive control and the development of disruptive drug-stimuli associations that can drive behavior. A reason for the strong influence drugs of abuse can exert on cognition may be the striking overlap between the neurobiological substrates of addiction and of learning and memory, especially areas involved in declarative memory. Declarative memories are critically involved in the formation of autobiographical memories, and the ability of drugs of abuse to alter these memories could be particularly detrimental. A key structure in this memory system is the hippocampus, which is critically involved in binding multimodal stimuli together to form complex long-term memories. While all drugs of abuse can alter hippocampal function, this review focuses on nicotine. Addiction to tobacco products is insidious, with the majority of smokers wanting to quit; yet the majority of those that attempt to quit fail. Nicotine addiction is associated with the presence of drug-context and drug-cue associations that trigger drug seeking behavior and altered cognition during periods of abstinence, which contributes to relapse. This suggests that understanding the effects of nicotine on learning and memory will advance understanding and potentially facilitate treating nicotine addiction. The following sections examine: 1) how the effects of nicotine on hippocampus-dependent learning change as nicotine administration transitions from acute to chronic and then to withdrawal from chronic treatment and the potential impact of these changes on addiction, 2) how nicotine usurps the cellular mechanisms of synaptic plasticity, 3) the physiological changes in the hippocampus that may contribute to nicotine withdrawal deficits in learning, and 4) the role of genetics and developmental stage (i.e., adolescence) in these effects.
1.0 Introduction
While addiction is often associated with reward, reinforcement, and changes in dopamine function, it is the premises of this review that a major contributing factor to the development and maintenance of addiction is the ability of substances of abuse to usurp the neurobiological substrates of learning and cellular plasticity and thus exert strong and lasting influences on behavior. Tremendous overlap exists between the neural substrates of learning and memory and the neural substrates of addiction. Areas that show overlap include, but are not limited to, the cerebral cortex, hippocampus, amygdala, and striatum (Kelley, 2004). In support, strong activation of memory-related brain regions that include the dorsolateral prefrontal cortex and hippocampus has been correlated with smoking-related cues in adult heavy smokers (Franklin, Wang, Wang, Sciortino, Harper, Li, Ehrman, Kampman, O’Brien, Detre, and Childress, 2007). Further, a similar effect has also been observed in adolescent light smokers (Rubinstein, Luks, Moscicki, Dryden, Rait, and Simpson, 2011), which is indicative of the strong contribution memory can make to addictive processes very early on in the development of addiction. In addition, another study found that smoking-related images increased activation of the amygdala (an area involved in emotion-based learning (LeDoux, Thompson, Iadecola, Tucker, and Reis, 1983; Phillips and LeDoux, 1992; Sarter and Markowitsch, 1985)) and hippocampus (Due, Huettel, Hall, and Rubin, 2002). The striatum is involved with reward (Delgado, 2007) and reward-related learning (Belin, Jonkman, Dickinson, Robbins, and Everitt, 2009), and years of smoking are associated with reduced reward-related activity in the striatum (Rose, Ross, Salmeron, Lee, Shakleya, Huestis, and Stein, 2012). Together, these brains regions are often involved either directly (prefrontal cortex (Blumenfeld and Ranganath, 2007) and hippocampus (Scoville and Milner, 1957)) or indirectly (amygdala: emotional modulation of memories (Dere, Pause, and Pietrowsky, 2010) and striatum: reward-based memories and decision making (Marschner, Mell, Wartenburger, Villringer, Reischies, and Heekeren, 2005)) in declarative and episodic memory processes. Because declarative and episodic memory systems support the acquisition and maintenance of memories of life events and experiences that contribute to the definition of the self (Tulving, 1972), the ability of drugs of abuse to alter this system may be one factor why drugs of abuse can exert such a strong control on behavior and become a central focus of the addict (Gould, 2010). This pathological usurpation of the learning and memory machinery can easily lead to increased preoccupation with drug-related stimuli and memories, and progressively less interest in societal, familial, and work-related issues (DSM-IV-TR, 2000). Because the hippocampus is critically involved in the formation of long-term declarative and episodic memories, this review will focus on how nicotine alters hippocampus-dependent learning and the underlying neural substrates, how these effects change with the duration of nicotine treatment, and how these changes in learning and memory could contribute to nicotine addiction.
2.0 Contribution of the Hippocampus to Cognitive Processes
The hippocampus is critically involved in memory formation and retrieval as well as the processing of emotionally relevant information. In order to fully appreciate the impact of nicotine on hippocampal function it is important to understand how the anatomy and the afferent and efferent connections of the hippocampus contribute to its unique role in learning and memory. The hippocampus’ physical location places it in within the Papez circuit (Papez, 1937), now known as the limbic system (MacLean, 1949; MacLean, 1952). The limbic system is made up of the hippocampus, hypothalamus, the septal nucleus, the amygdala, and the anterior cingulate cortex. MacLean made great strides in interpreting the limbic system as more than “subcortical” structures, emphasizing that it is critically important for the affective processing of information. A striking quote from MacLean’s 1949 paper states “the hippocampal formation provides the kind of analyzer that can derive universals from the particulars of experience and relate them symbolically in the experience of emotion”. This statement is an amazingly apt description for the way the hippocampus and its substructures process information for the formation and retrieval of memory, even though MacLean was referring to its contribution in emotional processing. The work of Papez and MacLean correctly noted that the hippocampus was located within a network that allowed it to communicate with both subcortical and cortical structures and ultimately direct the behavior of the organism.
The idea that the hippocampus plays a role in the formation and storage of memories was greatly advanced with Scoville and Milner’s pioneering work on patient H. M.. Patient H. M. suffered from severe epilepsy and a radical treatment bilaterally resected portions of his medial temporal lobe, including the entire hippocampal formation. Scoville and Milner (1957) documented that without an intact hippocampal region, new declarative memories (i.e., facts and experiences) could not be formed (a condition known as anterograde amnesia). H. M.’s amnesia included both semantic (memory for events) and episodic (autobiographical) memories, as defined later by Endel Tulving (1972). In the previously mentioned study, patients with less severe damage (i.e., limited to the hippocampus itself) also had pronounced memory deficits, although these were less severe than those of H. M.. Interestingly, it appears as though the patients with lesions limited to the hippocampus were able to (if somewhat poorly) remember autobiographical details, but were unable to recall recently learned associations or to remember/recognize drawings and stories they just learned. Together, these findings set in motion the identification of the hippocampus as a memory-related brain structure, an idea that dominates the field today. It is now well know that one of the main functions of the hippocampus is to consolidate short-term declarative memories into long-term memories, which then can be stored in a distributed network in the cortex (Bontempi, Laurent-Demir, Destrade, and Jaffard, 1999; Maviel, Durkin, Menzaghi, and Bontempi, 2004; McClelland, McNaughton, and O’Reilly, 1995; Squire, 1992; Squire and Alvarez, 1995). Another role the hippocampus plays in memory is the binding of configural representations such that the organism can learn that two or more stimuli may have different meanings when they are presented alone or in combination (Rudy and Sutherland, 1989). This ability to form configural representation may be one reason why the hippocampus is critical for spatial navigation (O’Keefe and Dostrovsky, 1971) and contextual learning (Kim and Fanselow, 1992; Phillips and LeDoux, 1992); processes that require the formation of multimodal associations. A capacity of nicotine to modulate long-term configural memory formation could have serious and long-lasting effects on behavior.
Since the early work of Ramón y Cajal (1911), it has been known that the hippocampus proper is made up of subregions including the dentate gyrus (DG) and the areas of Ammon’s horn (CA1, CA2, and CA3), which receive innervation from the entorhinal cortex (figure 1). Behaviorally, studies of the hippocampus have often either treated the structure as a whole or have focused on delineating the specific functions of its subregions (i.e., DG, CA1–CA3). The hippocampal subregions play an important role in the function of the hippocampal network by filtering, amplifying, and associating discrete neural events. The efferent and afferent projections of each region differ, and they together comprise a circuit that begins in the entorhinal cortex and ends with projections to the entorhinal cortex and subiculum (Andersen, Bland, and Dudar, 1973; Bartesaghi, Gessi, and Migliore, 1995). Understanding the circuitry of the hippocampus is important as it is yet unknown if nicotine modulates learning and memory through acting at specific subregions or throughout the entire hippocampus.
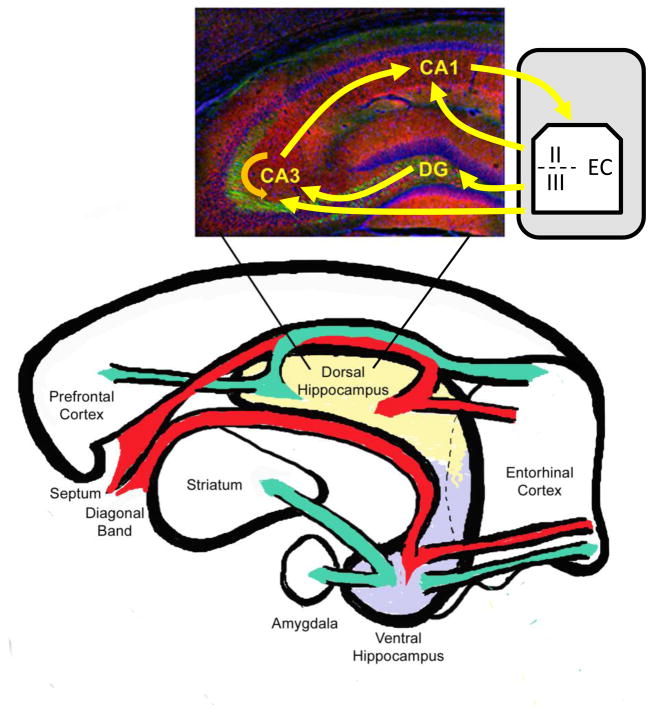
Schematic drawing of the hippocampal circuitry. Neural information flows in a loop from the entorhinal cortex through the subfields of the hippocampus and back to the entorhinal cortex as well as to the prefrontal cortex, amygdala, and striatum (specifically the nucleus accumbens). Dorsal and ventral hippocampi have distinct circuitry with the dorsal hippocampus projecting primarily to the prefrontal cortex and the ventral hippocampus projecting primarily to the amygdala and nucleus accumbens and this likely contributes to their functional differences. Hippocampal afferents are shown in red, while hippocampal efferents are shown in green. Inset image indicates the basic trisynaptic (EC-DG-CA3-CA1), disynaptic (EC-CA3-CA1) and monosynaptic (EC-CA1) information streams using yellow arrows and the CA3 recurrent collaterals using an orange arrow.
2.1 Dentate Gyrus
The DG receives afferent projections via the perforant path from layer II of the entorhinal cortex (van Groen, Miettinen, and Kadish, 2003), which contains grid cells and head location cells whose output can ultimately be transduced into hippocampal place cell firing (Moser, Kropff, and Moser, 2008). The dentate gyrus feeds information forward to CA3 proximal dendrites (Ribak, Seress, and Amaral, 1985) through unmyelinated axons called mossy fibers (for review, see Amaral, Scharfman, and Lavenex, 2007). Much research has focused on determining the behavioral contributions that the dentate gyrus makes to learning and memory. Numerous studies have shown that the DG is critical for spatial reference memory (Barbosa, Pontes, Ribeiro, Ribeiro, and Silva, 2012; Beselia, Maglakelidze, Chkhikvishvili, Burjanadze, and Dashniani, 2010; Gilbert, Kesner, and Lee, 2001; Hernandez-Rabaza, Hontecillas-Prieto, Velazquez-Sanchez, Ferragud, Perez-Villaba, Arcusa, Barcia, Trejo, and Canales, 2008; Hunsaker, Mooy, Swift, and Kesner, 2007; Jeltsch, Bertrand, Lazarus, and Cassel, 2001; Lee, Hunsaker, and Kesner, 2005a; Xavier, Oliveira-Filho, and Santos, 1999) and spatial working memory (Babar, Melik, and Ozgunen, 2002; Babar, Melik, Ozgunen, and Polat, 2002; Costa, Bueno, and Xavier, 2005; Hernandez-Rabaza, Barcia, Llorens-Martin, Trejo, and Canales, 2007; Jeltsch et al., 2001; Niewoehner, Single, Hvalby, Jensen, Meyer zum Alten Borgloh, Seeburg, Rawlins, Sprengel, and Bannerman, 2007; Xavier et al., 1999). In addition, the DG is involved in contextual learning and memory; manipulations that impair DG function lead to deficits in contextual memory and context discrimination (Daumas, Ceccom, Halley, Frances, and Lassalle, 2009; Hernandez-Rabaza et al., 2008; Lee and Kesner, 2004; McHugh, Jones, Quinn, Balthasar, Coppari, Elmquist, Lowell, Fanselow, Wilson, and Tonegawa, 2007). Further, optogenetic activation of neurons previously active during contextual fear conditioning induce a fear response in a novel context free of fearful associations (Liu, Ramirez, Pang, Puryear, Govindarajan, Deisseroth, and Tonegawa, 2012). These studies, taken together, suggest that DG activity is both necessary and sufficient for expression of contextual fear conditioning. There is also some evidence for a role of DG in processing temporal information (Costa et al., 2005). Overall, there is strong support for DG involvement in processing spatial and contextual information.
2.2 CA3
The CA3 region of the hippocampus receives afferent projections from the DG granule neurons and the entorhinal cortex. CA3 receives afferent projections directly from layer II of the entorhinal cortex in addition to the information it receives from the DG (which also receives information from layer II of the entorhinal cortex); this is also referred to as the monosynaptic projection from the entorhinal cortex (Wu and Leung, 1998). The DG projections synapse at the proximal dendrites (Ribak et al., 1985) while the entorhinal cortex/perforant pathway projections synapse at the apical dendrites. It is possible that the information reaching CA3 indirectly from entorhinal cortex via the DG is strengthened by the monosynaptic (direct) connection from entorhinal cortex to CA3 and this may facilitate changes in synaptic plasticity. The CA3 subfield of the hippocampus has a multitude of recurrent collaterals, where neurons synapse on the region’s own dendrites. This unique structural organization of CA3 allows it to function as a powerful auto-associative network (Bennett, Gibson, and Robinson, 1994), with each neuron synapsing on several nearby CA3 neurons within and across cerebral hemispheres (Ishizuka, Weber, and Amaral, 1990; Li, Somogyi, Ylinen, and Buzsaki, 1994). This recurrent activation thereby increases the strength of neuronal activation and synchronizes CA3 neuronal activity, which may allow associations to occur between various sources of incoming information.
CA3 is involved in similar learning and memory processes as the DG. Specifically, CA3 has been shown to be important for spatial working memory (Gilbert and Kesner, 2006; Lee, Jerman, and Kesner, 2005b) and spatial reference memory (Barbosa et al., 2012; Florian and Roullet, 2004; Handelmann and Olton, 1981; Holahan and Routtenberg, 2011; Hunsaker et al., 2007; Jerman, Kesner, and Hunsaker, 2006; Kesner, Hunsaker, and Warthen, 2008; Lassalle, Bataille, and Halley, 2000; Lee et al., 2005a; Nakazawa, Quirk, Chitwood, Watanabe, Yeckel, Sun, Kato, Carr, Johnston, Wilson, and Tonegawa, 2002; Nakazawa, Sun, Quirk, Rondi-Reig, Wilson, and Tonegawa, 2003; Roozendaal, Phillips, Power, Brooke, Sapolsky, and McGaugh, 2001; Steffenach, Sloviter, Moser, and Moser, 2002; Stubley-Weatherly, Harding, and Wright, 1996; Stupien, Florian, and Roullet, 2003). In addition, CA3 activity is critical for the acquisition and consolidation of contextual memory and context discrimination (Cravens, Vargas-Pinto, Christian, and Nakazawa, 2006; Daumas et al., 2009; Daumas, Halley, Frances, and Lassalle, 2005; Daumas, Halley, and Lassalle, 2004; Lee and Kesner, 2004; McHugh and Tonegawa, 2009). Finally, CA3 is important for memory of spatial/temporal sequences (Lee et al., 2005b; Li and Chao, 2008). Because CA3 is implicated in many of the same forms of memory as the DG, this may allow the intact hippocampal circuit to strengthen and reinforce these mnemonic processes.
CA3 axons project to ipsilateral CA1 through axons termed the Schaffer collaterals (Ishizuka et al., 1990) and to contralateral CA1, CA2, and CA3 via the commissural collaterals (Blackstad, 1956; Fricke and Cowan, 1978). The Schaffer collaterals along with the commissural collaterals are important pathways for neural plasticity involved in learning and memory (Bliss and Collingridge, 1993). The Schaffer collaterals likely facilitate consolidation of associations that were both processed and strengthened by the DG and CA3 regions of the hippocampus. Other efferent projections of the CA3 region of the hippocampus are to the lateral septal nucleus and the nucleus of the diagonal band as well as to known reward-related neural areas including the nucleus accumbens and the medial forebrain bundle (Raisman, Cowan, and Powell, 1966).
2.3 CA2
CA2 has received much less attention compared to the other subdivisions of the hippocampus. CA2 pyramidal neurons receive input from layer II and III of the entorhinal cortex (Chevaleyre and Siegelbaum, 2010) as well as the supramammillary nucleus of the hypothalamus (Borhegyi and Leranth, 1997; Magloczky, Acsady, and Freund, 1994), the medial septal nuclei, the vertical and horizontal limbs of the nucleus of the diagonal band of Broca, the median raphe nucleus (Cui, Gerfen, and Young, 2012), and intrahippocampal projections from CA3 (Ishizuka et al., 1990). CA2 neurons project bilaterally to CA1 and CA3 and contralaterally to CA2, as well as sending projections to the medial and lateral septal nuclei, vertical and horizontal limbs of the diagonal band of Broca, and the supramammillary nucleus (Cui et al., 2012). One potential reason for the limited studies of CA2 may be a lack of a clear evidence for the involvement of CA2 in hippocampal plasticity. It is interesting that compared to other areas of the hippocampus, CA2 Schaffer collaterals are remarkably resistant to the induction of synaptic plasticity such as long-term potentiation (LTP) (Zhao, Choi, Obrietan, and Dudek, 2007); though, select drugs such as caffeine may be able to potentiate synaptic plasticity specifically in this region (Simons, Caruana, Zhao, and Dudek, 2011). While CA2 may not be critically involved in synaptic plasticity, disrupted cellular structure is observed in area CA2 of patients with schizophrenia and bipolar disorder (Benes, Kwok, Vincent, and Todtenkopf, 1998). This suggests that changes to this area may contribute to some of the symptoms of these disorders and that this area may deserve future research.
2.4 CA1
CA1 receives afferent projections from CA3, as mentioned previously, but also contains a direct projection from layer III of the entorhinal cortex (Empson and Heinemann, 1995). It is interesting to note that the lateral entorhinal cortex may respond primarily to object/location information while the medial entorhinal cortex may respond preferentially to spatial information (Deshmukh and Knierim, 2011). Further, there appears to be a distinct organization to the entorhinal-CA1 projections, such that the spatial information from the medial entorhinal cortex reaches the proximal part of CA1 (i.e., near CA2) while the lateral entorhinal cortex sends information to the more distal portions of CA1 (i.e., near subiculum) (Henriksen, Colgin, Barnes, Witter, Moser, and Moser, 2010). The lateral entorhinal cortex likely relays object-related information (Zhu, Brown, and Aggleton, 1995).
The critical importance of region CA1 for normal mnemonic processes is exemplified when examining humans with lesions localized to the CA1 region of the hippocampus. Briefly, deficits are observed in forming new declarative memories, but subjects with CA1 lesions have intact memory for previously learned material (Zola-Morgan, Squire, and Amaral, 1986). Examples of processes that can be disrupted by CA1 lesions/inactivations include temporal processes (Gilbert et al., 2001; Kesner, Hunsaker, and Ziegler, 2011), spatial memory (Hunsaker et al., 2007; Lee et al., 2005b; Lu, Jia, Janus, Henderson, Gerlai, Wojtowicz, and Roder, 1997; Ridley, Timothy, Maclean, and Baker, 1995; Stubley-Weatherly et al., 1996; Tonegawa, Tsien, McHugh, Huerta, Blum, and Wilson, 1996; Tsien, Huerta, and Tonegawa, 1996), and contextual memory (Daumas et al., 2005; Lee and Kesner, 2004; Lu et al., 1997; Misane, Kruis, Pieneman, Ogren, and Stiedl, 2013). However, not all studies report a critical involvement of CA1 in these processes. Specifically, some inactivation studies reported no involvement of CA1 in temporal, spatial, and contextual processes (Barbosa et al., 2012; Daumas et al., 2009) and another study found that lesions of entorhinal cortex projections to CA1 did not disrupt spatial memory 24 hours after training, but did disrupt performance 4 weeks later (Remondes and Schuman, 2004).
CA1 is the major source of hippocampal efferents to subcortical structures through the subiculum (Canteras and Swanson, 1992; Groenewegen, Vermeulen-Van der Zee, te Kortschot, and Witter, 1987; Ishizuka, 2001; Swanson and Cowan, 1975; Witter and Groenewegen, 1990; Witter, Ostendorf, and Groenewegen, 1990) and to the cortex via the entorhinal cortex (Agster and Burwell, 2009; Burwell and Amaral, 1998; Swanson and Kohler, 1986). The anterior (i.e., dorsal) portion of CA1 projects to the anterior thalamic nuclei and the medial and lateral mammillary nuclei (Raisman et al., 1966), and the posterior (i.e., ventral) portion of CA1 projects to the septofimbrial nuclei, medial septal nuclei, diagonal band nuclei, ventromedial lateral septal nucleus, nucleus accumbens (contralateral), anterior thalamic nuclei, and mamillary nuclei (Raisman et al., 1966). Additional anatomical tracing studies have revealed that the CA1 region of the hippocampus projects to the prefrontal cortex as well (Jay and Witter, 1991; Swanson, 1981). Moreover, the dorsal hippocampal CA1 region projects to the tenia tecta (Cenquizca and Swanson, 2007), a region that has recently been implicated in reward learning (Maddux and Holland, 2011) and the retrosplenial area, which has been implicated in reward processing (Ikemoto, Witkin, and Morales, 2003) and reward memory (Smith, Barredo, and Mizumori, 2012) as well as allocentric spatial memory (Vann and Aggleton, 2002) and spatial working memory (Keene and Bucci, 2009). Projections from the hippocampus to reward-related neural structures may be involved in the learning that occurs during the development of addiction.
In summary, the entorhinal cortex sends projections to the dentate gyrus, which processes the neural signals and projects to CA3. CA3 also receives projections from the entorhinal cortex. In CA3, direct entorhinal cortical projections and DG projections may be combined or compared prior to projecting to CA1. Finally, the information flowing from CA3 to CA1 can be compared to or combined with the direct input from the entorhinal cortex to CA1 (Yeckel and Berger, 1990). The overall outcome is likely to be the refinement and/or strengthening of the signal and the association of distinct neural signals into one neural representation. Neuronal tracing studies reveal that entorhinal cortex layer II cells project to the dentate gyrus and CA3 while layer III cells project to CA1 (van Groen et al., 2003); these inputs may either compete with or complement each other in order to refine the information stream. Interestingly, the amygdala innervates layer III of the ventrolateral part of the entorhinal cortex (Pikkarainen, Ronkko, Savander, Insausti, and Pitkanen, 1999); this may allow emotional content and context to modulate CA1 activity and plasticity. The convergence of multiple streams of entorhinal cortical input into DG, CA3, and CA1 may facilitate the amplification and strengthening of neural signals involved in declarative memory formation. An important and unresolved issue is how and where nicotine is modulating this circuitry.
2.5 Differences along the Dorsal/Ventral Axis of the Hippocampus
In the previous section the hippocampus was presented as a homogenous structure with discrete subregions such as the dentate gyrus, CA3, and CA1, however, it is increasing becoming clear that the hippocampus is a heterogeneous neural structure divided into dorsal and ventral poles. The dorsal/ventral topography of the hippocampus is evident with differences in neurochemical function and associated behaviors, and in the sources of afferent fibers. Evidence is mounting that the dorsal hippocampus is more closely associated with spatial learning tasks, while the ventral hippocampus is more related to emotion processing (Fanselow and Dong, 2010; Moser and Moser, 1998). We will describe these dorsal-ventral differences in general terms and then integrate this into our model of how these differences may explain some of the effects of nicotine on learning.
The dorsal hippocampus has been extensively shown to be involved in learning and memory paradigms that require the use of temporal, spatial, and working memory. Dorsal hippocampal lesions and inactivation produce deficits in tasks that require temporal processing such as trace eyeblink and fear conditioning and memory for sequences (Compton, 1993; Czerniawski, Ree, Chia, and Otto, 2012; Czerniawski, Yoon, and Otto, 2009; Esclassan, Coutureau, Di Scala, and Marchand, 2009; Lee et al., 2005b; Takehara, Kawahara, Takatsuki, and Kirino, 2002). The dorsal hippocampus is also critical for spatial and contextual learning and memory (Anagnostaras, Maren, and Fanselow, 1999; Czerniawski et al., 2012; Esclassan et al., 2009; Ferbinteanu, Ray, and McDonald, 2003; Flavell and Lee, 2012; Kim and Fanselow, 1992; Loureiro, Lecourtier, Engeln, Lopez, Cosquer, Geiger, Kelche, Cassel, and Pereira de Vasconcelos, 2012; Maren, Aharonov, and Fanselow, 1997; Sannino, Russo, Torromino, Pendolino, Calabresi, and De Leonibus, 2012; Schroeder, Wingard, and Packard, 2002; Wang, Finnie, Hardt, and Nader, 2012). Finally, the dorsal hippocampus has been shown to be important for working memory (especially spatial working memory) (Dzidzishvili, Ungiadze, and Davituliani, 1975; Izaki, Takita, and Akema, 2008; Lee et al., 2005b; Lee and Kesner, 2003; McHugh, Niewoehner, Rawlins, and Bannerman, 2008; Sannino et al., 2012; Stevens and Cowey, 1973).
At a behavioral level, ventral hippocampal inactivation/lesions alter affective processes. Specifically, ventral hippocampal disruption severely reduces the expression of fear behaviors (Bannerman, Grubb, Deacon, Yee, Feldon, and Rawlins, 2003; Bast, Zhang, and Feldon, 2001; Czerniawski et al., 2012; Czerniawski et al., 2009; Esclassan et al., 2009; Gilmartin, Kwapis, and Helmstetter, 2012; Kjelstrup, Tuvnes, Steffenach, Murison, Moser, and Moser, 2002; Maren and Holt, 2004; McEown and Treit, 2009; 2010; Pentkowski, Blanchard, Lever, Litvin, and Blanchard, 2006; Sierra-Mercado, Padilla-Coreano, and Quirk, 2011). The effects of ventral hippocampal disruption on the expression of fear is not limited to contextual and/or trace conditioning paradigms (the way dorsal hippocampal disruption is), but is pervasive in fear paradigms ranging from contextual/trace to predatory odor and auditory cue-invoked fear tasks as well as unconditioned fear. This suggests a specific role in emotion-charged memory or expression of emotion. In addition to evidence for ventral hippocampal involvement in fear expression, there is also evidence for its involvement in other anxiety/emotion-related behaviors. For instance, ventral hippocampal lesions reduce anxiety in an elevated T-maze (Trivedi and Coover, 2004). Further, increased ventral hippocampal activity drives activity in the nucleus accumbens (Bardgett and Henry, 1999; Bast, Zhang, Heidbreder, and Feldon, 2001), a region critically involved in locomotion and drug reward-related neural activity (Mansvelder, De Rover, McGehee, and Brussaard, 2003). There is evidence that the ventral hippocampus may be important for some forms of memory retrieval (i.e., Morris water maze and radial arm maze) (Ferbinteanu et al., 2003; Loureiro et al., 2012; Stubley-Weatherly et al., 1996), but the majority of evidence points to a more specific role of the ventral hippocampal in processing of affective information.
Differences in learning and memory processes mediated by dorsal and ventral hippocampi may be related to differences in synaptic plasticity between the two regions. Specifically, robust LTP is seen in the dorsal hippocampus while more modest LTP is found in the ventral hippocampus (Colgin, Kubota, Jia, Rex, and Lynch, 2004; Papatheodoropoulos and Kostopoulos, 2000). Further, the modulation of hippocampal LTP may even differ across dorsal and ventral regions, such that low basal levels of ventral hippocampal LTP is enhanced by stress via mineralocorticoid receptor activity, while high basal levels of dorsal hippocampal LTP is disrupted by stress via glucocorticoid receptor activity (Maggio and Segal, 2007a). Further, Group I metabotropic glutamate receptors mediate changes in ventral hippocampal, but not dorsal hippocampal, synaptic plasticity (Maggio and Segal, 2007b). These differences in synaptic plasticity in dorsal and ventral hippocampus may underlie some of the distinct behavioral functions associated with dorsal versus ventral hippocampus.
Differences in the dorsal versus ventral hippocampal afferent projections may contribute to the functional differences between the areas (figure 1). Different regions of the entorhinal cortex project to the dorsal versus ventral hippocampus (Ruth, Collier, and Routtenberg, 1982; 1988; van Groen et al., 2003). Specifically, the dorsal hippocampus receives projections from posterior regions of the medial entorhinal area (Ruth et al., 1982) and a rostrocaudal strip of neurons within the dorsolateral area of the lateral entorhinal cortex (Ruth et al., 1988). In contrast, the ventral hippocampus receives projections from ventral and anterior/medial regions of the medial entorhinal area (Ruth et al., 1982) and from caudomedial portions of the lateral entorhinal cortex (Ruth et al., 1988). In support of a functional consequence to the topographical organization of projections from the entorhinal cortex to the hippocampus, recent evidence points to a select portion of the medial entorhinal cortex being critical for spatial information processing, specifically the dorsolateral band (Fyhn, Molden, Witter, Moser, and Moser, 2004). Specifically, selective lesions of the dorsolateral band of the medial entorhinal cortex, which projects to the dorsal hippocampus, disrupted spatial memories, while lesions of the ventromedial band, which projects to the ventral hippocampus, disrupted anxiety-related behaviors (Steffenach, Witter, Moser, and Moser, 2005).
The dorsal and ventral hippocampi are also differentially innervated by subregions of the septum as well. Within the basal forebrain, the medial septal nucleus and the horizontal diagonal band are the main sources of hippocampal cholinergic projections. Within the hippocampus, the septum projects to the supragranular regions of the dentate gyrus and the CA3/4 pyramidal regions of Amon’s horn (Mosko, Lynch, and Cotman, 1973; Raisman, 1966) with modest projections to the CA1 pyramidal region (Crutcher, Madison, and Davis, 1981). Further analysis revealed a topographical organization; the vertical limb of the diagonal band and the medial septal nucleus projects to the dorsal hippocampus while both the horizontal and vertical limbs of the diagonal band and the intermediolateral septum project to the ventral hippocampus (Amaral and Kurz, 1985; Meibach and Siegel, 1977). There are also reports of modestly higher levels of acetylcholine in the ventral hippocampus, which may contribute to differences in function between the dorsal and ventral areas (Hoover, Muth, and Jacobowitz, 1978).
Further differences in the cholinergic processes of the dorsal and ventral hippocampi have been reported. For example, dorsal hippocampal acetylcholine levels were positively correlated with hippocampal theta rhythm amplitude, while ventral hippocampal acetylcholine levels were not (Monmaur, Collet, Puma, Frankel-Kohn, and Sharif, 1997). It has been suggested that the hippocampal theta rhythm subserves the processing of spatial information (Hasselmo, 2005); the ability of acetylcholine to modulate theta rhythms in the dorsal hippocampus may be one mechanism that supports spatial information processing. In addition, there may not be a substantial theta rhythm in the ventral hippocampus, which may reflect the decreasing role that the ventral hippocampus plays in spatial processing (Royer, Sirota, Patel, and Buzsaki, 2010). Additional dorsal-ventral differences exist in response to administration of cholinergic drugs. Galanin, a modulator of acetylcholine release, stimulated dorsal hippocampal acetylcholine release while it inhibited ventral hippocampal acetylcholine release (Ogren, Schott, Kehr, Yoshitake, Misane, Mannstrom, and Sandin, 1998; Yoshitake, Yoshitake, Savage, Elvander-Tottie, Ogren, and Kehr, 2011). Furthermore, low dose nicotine infusions directly into the dorsal hippocampus increased norepinephrine levels in the dorsal hippocampus while nicotine infused into the ventral hippocampus reduced norepinephrine levels (Shearman, Rossi, Sershen, Hashim, and Lajtha, 2005).
Due to the differences in neurochemical properties, electrophysiological properties, and innervation patterns of the dorsal and ventral hippocampi, it is becoming increasingly apparent that the dorsal and ventral hippocampi are distinct in terms of both their structure and function. There are numerous other dorsal-ventral differences not reviewed here including differences in patterns of gene expression; for an excellent review see Fanselow and Dong (2010). We will provide evidence later in this review for specific effects of nicotine on hippocampal function, and how these effects differ across the dorsal-ventral axis of the hippocampus. Understanding these differences and understanding where nicotine acts in the hippocampus will aid in understanding how nicotine affects behavior, which will be reviewed in section 5.1.
2.6 Hippocampal Synaptic Plasticity
Changes in the strength of synapses likely underlie changes in network dynamics that store neural representations of experiences (i.e., memory) (Hebb, 1949; Sherrington, 1906). By far the most extensively studied model of the cellular changes that may underlie learning and memory is LTP of hippocampal synapses (for review see Bliss and Collingridge, 1993). Briefly, in the prototypical model of LTP, electrical stimulation leads to long lasting potentiation of synaptic efficacy (Bliss and Gardner-Medwin, 1973; Bliss and Lomo, 1973). When both cellular depolarization and glutamatergic neurotransmission occur, N-Methyl-D-aspartic acid (NMDA) receptors act as coincidence detectors on the postsynaptic terminal because they require concurrent depolarization and ligand (glutamate) binding in order to activate their ion-channel properties (Bliss and Collingridge, 1993). Under resting conditions, NMDA receptors have a Mg2+ ion that fits in their ionic pore, blocking conductance even in the presence of the endogenous ligand glutamate (Ascher and Nowak, 1988). When the postsynaptic terminal is depolarized by α-amino-3-hydroxy-5-methyl-4-isoxazolepropionic acid (AMPA) receptor activation (Collingridge, Kehl, and McLennan, 1983), this change in membrane potential can relieve the Mg2+ block from the NMDA receptor and allow glutamate-triggered Ca2+ influx, which is critical for the development of many forms of LTP (Lynch, Larson, Kelso, Barrionuevo, and Schottler, 1983). Increases in intracellular calcium levels contribute to enhanced synaptic efficacy by inducing changes in protein activation (Akers, Lovinger, Colley, Linden, and Routtenberg, 1986; Klann, Chen, and Sweatt, 1991), mRNA synthesis (Frey, Frey, Schollmeier, and Krug, 1996; Nguyen, Abel, and Kandel, 1994; Wiegert, Hofmann, Bading, and Bengtson, 2009), and protein translation (Frey, Krug, Reymann, and Matthies, 1988; Krug, Lossner, and Ott, 1984; Otani, Marshall, Tate, Goddard, and Abraham, 1989). It is thought that this cascade of events is a cellular mechanism of memory storage that drives the changes in synaptic plasticity that underlies learning.
There are many intracellular mechanisms that have been shown to be critical to learning and memory (Platenik, Kuramoto, and Yoneda, 2000; Poser and Storm, 2001) and similar mechanisms may be important for addiction as well (for reviews see Berke and Hyman, 2000; Nestler, 2002). For the purposes of this review we will focus on the canonical pathway leading from Ca2+ influx to CREB-dependent gene transcription, and how nicotine may usurp these processes in order to strengthen addiction. The CREB-dependent gene transcription pathway is most often thought of as being activated by initial transient increases in intracellular Ca2+, which is produced by NMDA receptor activation. This Ca2+ influx activates adenylyl cyclase to convert intracellular adenosine diphosphate (ADP) to cyclic adenosine monophosphate (cAMP) (Poser and Storm, 2001). Increasing the concentration of cAMP subsequently activates protein kinase A (PKA), which has been critically implicated in long-term memory formation (Abel and Nguyen, 2008). Protein kinase A then phosphorylates cAMP-response element binding protein (CREB), leading to changes in gene transcription and the translation of new proteins that may support the storage of long-term memory (Impey, Smith, Obrietan, Donahue, Wade, and Storm, 1998b; Silva, Kogan, Frankland, and Kida, 1998). There is also evidence that the mitogen activated protein kinase (MAPK) signaling pathway may be activated by PKA and may also contribute to the phosphorylation of CREB necessary for neuronal storage of long-term memory (Impey, Obrietan, Wong, Poser, Yano, Wayman, Deloulme, Chan, and Storm, 1998a). In support of this general cascade of events being critical to synaptic plasticity, it has been extensively shown that these molecules are critical for the formation of LTP. For instance, mice that lack functional adenylyl cyclase (Wong, Athos, Figueroa, Pineda, Schaefer, Chavkin, Muglia, and Storm, 1999), PKA (Abel, Nguyen, Barad, Deuel, Kandel, and Bourtchouladze, 1997), or CREB (Bourtchuladze, Frenguelli, Blendy, Cioffi, Schutz, and Silva, 1994) all have selective deficits in LTP and long-term memory. Additionally, pharmacological inhibition of PKA (Bernabeu, Bevilaqua, Ardenghi, Bromberg, Schmitz, Bianchin, Izquierdo, and Medina, 1997; Huang and Kandel, 1994) or the prototypical MAPK, extracellular-regulated kinase 1/2 (ERK1/2), impair LTP and long-term memory (Atkins, Selcher, Petraitis, Trzaskos, and Sweatt, 1998; English and Sweatt, 1997). The ability of this signaling cascade, and other cascades, to regulate gene transcription and synthesis of new proteins is thought to be the key mechanism of long-term memory storage and the ability of acetylcholine and/or nicotine to modulate theses processes may impact memories.
3.0 Acetylcholine
While multiple neurotransmitters can regulate and modulate hippocampal plasticity, acetylcholine’s role in these processes is well established (for review see Hasselmo, 2006). Acetylcholine is a neurotransmitter able to modulate neural functioning and behavior. Acting as a neuromodulator, it can either boost excitatory drive or activate inhibitory neurons and dampen cellular activation (Hasselmo, 1995). The ability of cholinergic agonists to modulate neuronal activation may contribute to learning and memory by increasing the probability that neural activation will be sufficient to change synaptic plasticity. Brain acetylcholine receptors are divided into two classes based on selective and distinct binding of cholinergic agonists muscarine and nicotine. Muscarine binds exclusively to muscarinic acetylcholine receptors (mAChRs), while nicotine binds to nicotinic acetylcholine receptors (nAChRs) (for review see Graef, Schonknecht, Sabri, and Hegerl, 2011).
In the peripheral nervous system, acetylcholine acts on nAChRs located at neuromuscular junctions (Kemp, Morley, Dwyer, and Bradley, 1980) and mAChRs to regulate the parasympathetic nervous system (Heilbronn and Bartfai, 1978). In the brain, there are three main sources of acetylcholine that affect cognitive processes via muscarinic and nicotinic AChRs (for review see Graef et al., 2011). The main focus of this review will be the basal forebrain cholinergic projection, which releases acetylcholine to several areas of the cortex and hippocampal formation (Bigl, Woolf, and Butcher, 1982; Raisman, 1966; Woolf, Eckenstein, and Butcher, 1983; Woolf, Hernit, and Butcher, 1986). Other sources of acetylcholine include brainstem nuclei that project to cortical and subcortical regions (Woolf and Butcher, 1986) and the striatum, which produces its own cholinergic innervation (Woolf and Butcher, 1981; Zhou, Wilson, and Dani, 2002). The broad distribution of cholinergic receptors in the cortex and the limbic system (including the hippocampus) makes acetylcholine an ideal candidate for modulating cognitive functioning (Hasselmo, 2006; Hasselmo and Bower, 1993; Sarter and Bruno, 1997), and it has even been suggested to be the basis of “consciousness” (Perry, Walker, Grace, and Perry, 1999).
3.1 Muscarinic Acetylcholine Receptors
Studies that evaluated the localization of mAChRs in the hippocampus, using autoradiography and antibody-based techniques, revealed a high level of expression in the hippocampus with a uniform distribution throughout the dorsal-ventral length of the hippocampus (Levey, Kitt, Simonds, Price, and Brann, 1991; Spencer, Horvath, and Traber, 1986). Muscarinic acetylcholine receptors are seven-transmembrane/G-protein coupled receptors (7TM/GPCR) that can be excitatory or inhibitory (Cole and Nicoll, 1984; Valentino and Dingledine, 1981). Activation of these GPCRs (M1–M4) stimulates the dissociation of G proteins such as Gq/11 (Hassall, Stanford, Burnstock, and Buckley, 1993) from the receptors so that they can activate nearby ion channels (Brown, Abogadie, Allen, Buckley, Caulfield, Delmas, Haley, Lamas, and Selyanko, 1997; Caulfield, Robbins, Higashida, and Brown, 1993). The most common subtype of the receptor, the M1 subtype, is primarily found in the hippocampus (Graef et al., 2011). Although they play an established role in cognition, mAChRs have been extensively reviewed elsewhere (see Brown, 2010) and will only be briefly discussed here as they are not the focus of the current review.
3.2 Nicotinic Acetylcholine Receptors
Nicotinic acetylcholine receptors are pentameric ligand-gated ion channels. These receptors are distributed throughout the hippocampus with both pre and postsynaptic locations (Abood, Reynolds, Booth, and Bidlack, 1981; Clarke, Schwartz, Paul, Pert, and Pert, 1985; Costa and Murphy, 1983; Yoshida and Imura, 1979). Presynaptic nAChRs can stimulate neurotransmitter release (Araujo, Lapchak, Collier, and Quirion, 1988; Clarke and Reuben, 1996; Fabian-Fine, Skehel, Errington, Davies, Sher, Stewart, and Fine, 2001; Gray, Rajan, Radcliffe, Yakehiro, and Dani, 1996; Radcliffe, Fisher, Gray, and Dani, 1999; Wilkie, Hutson, Sullivan, and Wonnacott, 1996; Zarei, Radcliffe, Chen, Patrick, and Dani, 1999). Postsynaptic nAChRs likely contribute to neuronal depolarization (Alkondon, Pereira, and Albuquerque, 1996; Fabian-Fine et al., 2001; Zarei et al., 1999). Finally, nAChRs can also be found on both glutamatergic and GABAergic terminals in the hippocampus (Fabian-Fine et al., 2001; Radcliffe et al., 1999) where they can modulate excitatory and inhibitory processes. To better understand nACh processes, nAChR subtypes have to be examined because their function and location varies across subtype.
Acetylcholine binding to nAChRs leads to conformational changes in the receptors that alters their permeability to sodium (Na+) and Ca2+ ions (McKay, Placzek, and Dani, 2007). The various subunit composition of individual receptors dictates agonist binding affinity and ion permeability (e.g., Na+ and Ca2+) (Gotti, Clementi, Fornari, Gaimarri, Guiducci, Manfredi, Moretti, Pedrazzi, Pucci, and Zoli, 2009; Mihailescu and Drucker-Colin, 2000). Subunits that make up nAChRs in the brain can be either α (α2–10) or β (β2–4) and can form as heteromeric receptors (e.g., α4β2* where * denotes potential unknown subunit) or homomeric receptors (e.g., α7) that differ in their neurophysiological properties (for reviews see Alkondon and Albuquerque, 2004; Picciotto, Caldarone, King, and Zachariou, 2000). Additionally, various nAChR subtypes contain principal, complementary, or both principal and complementary acetylcholine binding sites, which means various subunit combinations can result in different numbers of overall acetylcholine binding sites. For example, the subunit has been identified as a binding site for nicotine (Dennis, Giraudat, Kotzyba-Hibert, Goeldner, Hirth, Chang, Lazure, Chretien, and Changeux, 1988; Middleton and Cohen, 1991), thus and number of subunits can determine the number of binding sites. Homomeric α7 receptors composed of five α7 subunits contain 5 acetylcholine binding sites; in comparison, heteromeric α4β2* receptors contain only 2 binding sites (Corringer, Le Novere, and Changeux, 2000; Le Novere, Corringer, and Changeux, 2002; Taly, Corringer, Guedin, Lestage, and Changeux, 2009). There is also evidence that α4/α4 junctions may form an additional agonist binding site whose contribution to agonist effects is still being elucidated (Mazzaferro, Benallegue, Carbone, Gasparri, Vijayan, Biggin, Moroni, and Bermudez, 2011). Finally, possible receptor localization can also include extra-synaptic locations such as glial cells (Gahring, Persiyanov, Dunn, Weiss, Meyer, and Rogers, 2004), where the mechanisms are less well studied.
The effects of nicotine at nAChRs are paradoxical. While nicotine is an agonist, it can inactivate nAChRs, which is due to the ability of nicotine to rapidly desensitize nAChRs (Hulihan-Giblin, Lumpkin, and Kellar, 1990; Sharp and Beyer, 1986). In addition, whereas chronic treatment with drugs of abuse such as cocaine and morphine can lead to receptor downregulation (Nader, Morgan, Gage, Nader, Calhoun, Buchheimer, Ehrenkaufer, and Mach, 2006; Volkow, Fowler, Wolf, Schlyer, Shiue, Alpert, Dewey, Logan, Bendriem, Christman, and et al., 1990; Werling, McMahon, and Cox, 1989), chronic nicotine treatment upregulates nAChRs (Marks, Burch, and Collins, 1983; Schwartz and Kellar, 1983). The functional down regulation of nAChRs due to desensitization has been hypothesized to be an important initial part of processes that lead to concurrent upregulation of nAChRs during chronic nicotine administration but not the only factor (Marks, 1999). In addition, it has been proposed that the desensitization and upregulation may contribute to tolerance and withdrawal (Dani and Heinemann, 1996; Gould, Portugal, Andre, Tadman, Marks, Kenney, Yildirim, and Adoff, 2012; Marks, Grady, and Collins, 1993; Wilkinson and Gould, 2013). Desensitization and upregulation, however, are not universal properties of all nAChRs. Factors that can influence these processes include nAChR localization and nAChR subunit composition. Upregulation of nAChRs associated with chronic nicotine treatment is greater in cerebral cortex and hippocampus compared to other areas such as thalamus (Marks, 1999). This regional specificity in upregulation may be related to differences in nAChR subtype expression and receptor composition in those regions. For instance, 4β2 nAChRs can be one of two stoichiometries: α43β22 or α42β23; however, the α43β22 stoichiometry has a lower sensitivity to nicotine, which may result in rapid desensitization while the α42β23 stoichiometry is associated with higher sensitivity to nicotine, which may result in a slower rate of desensitization (Kuryatov, Luo, Cooper, and Lindstrom, 2005; Nelson, Kuryatov, Choi, Zhou, and Lindstrom, 2003; Zwart and Vijverberg, 1998), and a greater degree of upregulation (Kuryatov et al., 2005; Moroni, Zwart, Sher, Cassels, and Bermudez, 2006; Nelson et al., 2003). These results suggest that desensitization is not the only factor contributing to receptor upregulation and perhaps the rate and/or duration of sensitization could contribute to receptor upregulation. Smoking may alter the sensitivity of the cholinergic system as nicotine administration led to the formation of more nAChRs with the α42β23 stoichiometry (Moroni et al., 2006; Nelson et al., 2003). In addition, 7 and 3β2 containing nAChRs showed faster rates of desensitization than 4β4 and 4β2 containing nAChRs (Chavez-Noriega, Crona, Washburn, Urrutia, Elliott, and Johnson, 1997), 6β2 and 4β2 showed similar desensitization but 6β2 nAChRs recovered faster (Xiao, Srinivasan, Drenan, Mackey, McIntosh, and Lester, 2011), and α4β2 receptors containing an α5 subunit were resistant to upregulation by chronic nicotine (Mao, Perry, Yasuda, Wolfe, and Kellar, 2008). In the hippocampus, differences between 7 nAChR and 4β2* nAChR desensitization and upregulation may contribute to some of the effects of nicotine on hippocampus-dependent processes.
3.3 Low-Affinity Homomeric α7 nAChR Localization in the Hippocampus
Nicotinic receptors can be largely categorized into high- and low-affinity based on relative binding of ligands such as nicotine and acetylcholine (Marks, Stitzel, Romm, Wehner, and Collins, 1986; Sershen, Reith, Lajtha, and Gennaro, 1981). A class of nAChRs exist that bind nicotine and acetylcholine with relatively low affinity (Amar, Thomas, Johnson, Lunt, and Wonnacott, 1993; Zhang, Vijayaraghavan, and Berg, 1994), but bind α-bungarotoxin with high affinity (Wonnacott, 1986). Evaluation of the α-bungarotoxin sensitive neuronal population of receptors revealed that they were, in fact, homomeric α7 nAChRs (Chen and Patrick, 1997) and that mice lacking α7 receptors lacked α-bungarotoxin binding (Orr-Urtreger, Goldner, Saeki, Lorenzo, Goldberg, De Biasi, Dani, Patrick, and Beaudet, 1997). 7 nAChRs show rapid desensitization (Olale, Gerzanich, Kuryatov, Wang, and Lindstrom, 1997) and upregulation, which returns to baseline quickly after cessation of nicotine treatment (Barrantes, Rogers, Lindstrom, and Wonnacott, 1995; Marks, Stitzel, and Collins, 1985; Rogers and Wonnacott, 1995). The anatomical distribution of nAChRs has also been evaluated, and there are different expression patterns across different brain regions. Homomeric α7 receptors are highly expressed in the granule and pyramidal cells of the hippocampus in addition to other limbic structures, such as the amygdala and hypothalamus (Dominguez del Toro, Juiz, Peng, Lindstrom, and Criado, 1994; Fabian-Fine et al., 2001; Seguela, Wadiche, Dineley-Miller, Dani, and Patrick, 1993). While the functions mediated by 7 nAChRs are not completely known, disregulation of 7 nAChRs may contribute to mental illnesses. In support of this, various genome-wide association studies (GWAS) have revealed that the α7 receptor has reduced expression in patients with schizophrenia (Freedman, Hall, Adler, and Leonard, 1995), driven by polymorphisms in the gene’s promoter region (Leonard, Gault, Hopkins, Logel, Vianzon, Short, Drebing, Berger, Venn, Sirota, Zerbe, Olincy, Ross, Adler, and Freedman, 2002). It is thought that reduced α7 receptor function is specifically important for the cognitive and sensory gating deficits observed in subjects with schizophrenia (Adler, Olincy, Waldo, Harris, Griffith, Stevens, Flach, Nagamoto, Bickford, Leonard, and Freedman, 1998). Nicotine administration can upregulate and desensitize low-affinity nAChRs (Barrantes et al., 1995; Marks et al., 1985; Olale et al., 1997; Rogers and Wonnacott, 1995). These effects may help alleviate symptoms associated with the altered gene expression observed in subjects with schizophrenia, which may contribute to the high rate of smoking observed in this patient population (Martin and Freedman, 2007; Olincy, Harris, Johnson, Pender, Kongs, Allensworth, Ellis, Zerbe, Leonard, Stevens, Stevens, Martin, Adler, Soti, Kem, and Freedman, 2006; Olincy and Stevens, 2007).
3.4 High-Affinity nAChR Localization in the Hippocampus
In addition to low-affinity nicotine binding sites throughout the hippocampus, there is also a high prevalence of distinct nAChR binding sites that bind acetylcholine (and nicotine) with high affinity (Marks and Collins, 1982; Marks et al., 1986). Comparing α4β2, α3β2, and α3β4 nAChRs, the high-affinity α4β2 receptors were identified as making up 99% of hippocampal nAChRs in the CA1 and DG regions of the hippocampus (Perry, Xiao, Nguyen, Musachio, Davila-Garcia, and Kellar, 2002). Other selective autoradiographic binding studies have revealed α2- and α3-containing receptors are present in the entorhinal cortex and α2-containing receptors are present in CA1 and CA3 of the hippocampus proper (Wada, Wada, Boulter, Deneris, Heinemann, Patrick, and Swanson, 1989). Genetic differences are also likely to affect the distribution of nAChRs in the brain. In support of this, Gahring and Rogers (2008) determined that the distribution of α4-containing nAChRs is dramatically different across 27 inbred mouse strains. Various nAChR subtypes may make unique contributions to nicotine addiction in various patient populations by altering sensitivity to reward, response to agonist, or by altering cognitive processing. 4β2* nAChRs desensitize at a slower rate than 7 nAChRs (Olale et al., 1997) and also show a longer lasting upregulation (Marks et al., 1985), which may be important for the effects of nicotine withdrawal on learning. In addition to their slow rate of desensitization and long-lasting upregulation, high-affinity nAChRs are attractive candidates for modulating cognition in that they are highly sensitive to agonist and gate a significant amount of Ca2+ (albeit lower than the α7 receptor) (Lax, Fucile, and Eusebi, 2002; Ragozzino, Barabino, Fucile, and Eusebi, 1998). Effects of high-affinity nAChR activation can include the induction of neurotransmitter release (Araujo et al., 1988; Wilkie et al., 1996) and postsynaptic depolarization (Alkondon et al., 1996; Zarei et al., 1999), and these may have dramatic effects on hippocampal function leading to alterations in learning and memory and addiction.
3.5 Acetylcholine and Learning
It is clear that cholinergic signaling has an important role in learning and memory. Lesions to the forebrain cholinergic projection system produced impairments in spatial and working memory paradigms (Hodges, Allen, Kershaw, Lantos, Gray, and Sinden, 1991a). Further, transplants of acetylcholine-rich brain tissue into the hippocampus of rats with forebrain cholinergic lesions ameliorated some of these deficits, indicating that acetylcholine in the hippocampus is critical to spatial and working memory task performance (Arendt, Allen, Marchbanks, Schugens, Sinden, Lantos, and Gray, 1989; Hodges et al., 1991a). In another set of studies, cholinergic agonists and antagonists were evaluated for their effects on memory in rats with basal forebrain lesions. Cholinergic antagonists (both nicotinic and muscarinic) impaired memory in lesioned rats at doses that were ineffective in disrupting sham lesioned animals. Additionally, nicotine reversed the deficits associated with basal forebrain cholinergic lesions (Hodges, Allen, Sinden, Mitchell, Arendt, Lantos, and Gray, 1991b). These studies provide strong evidence that acetylcholine is synthesized in the basal forebrain and acts on acetylcholine receptors in the hippocampus to regulate many forms of learning and memory.
Muscarinic and nicotinic cholinergic receptors are each important for cognition (Dilts, 1967), however, there may be dissociations in their relative efficacy at modulating memory and in the specific cognitive domain they affect. Muscarinic cholinergic signaling has been shown to be critical for successful long-term memory formation and retrieval (Deutsch, 1971), whereas nAChRs are often described as playing a more modulatory role in mediating cognitive processes (Mansvelder et al., 2003; Mansvelder, van Aerde, Couey, and Brussaard, 2006). Muscarinic cholinergic antagonists, such as scopolamine, produce amnesia for both short (Baron, Wright, and Wenger, 1998) and long term memories (Feiro and Gould, 2005; Gravius, Barberi, Schafer, Schmidt, and Danysz, 2006) supporting the involvement of mAChRs in memory formation. The evidence for a critical role of nAChRs in memory is more equivocal. For example, there are reports that mAChR antagonists produced a transient and profound amnesia, while antagonists at nAChRs produced a more subtle deficit (Gitelman and Prohovnik, 1992). Specifically, the muscarinic antagonist scopolamine was efficacious in disrupting alternation memory, short-term spatial memory, place discrimination memory, and both working and reference memory while the nAChR antagonist mecamylamine was not (Andrews, Jansen, Linders, and Princen, 1994; Clarke and Fibiger, 1990; Kikusui, Tonohiro, and Kaneko, 2000; Moran, 1993). In contrast to the previously mentioned reports, other studies provided evidence that mecamylamine produces deficits in spatial memory, working and reference memory, passive avoidance memory, and contextual memory performance (Decker and Majchrzak, 1992; Levin, McGurk, Rose, and Butcher, 1989; Riekkinen and Riekkinen, 1994; Vago and Kesner, 2007), but more modest systemic doses of mecamylamine did not disrupt contextual fear conditioning (Davis and Gould, 2006; Feiro and Gould, 2005; Gould and Lewis, 2005). Overall, muscarinic cholinergic mechanisms may be important for overt memory formation/retrieval, while nicotinic mechanisms may modulate memory processes.
4.0 Nicotine and Hippocampal Long-Term Potentiation
Nicotine can both facilitate and directly induce LTP. These effects of nicotine may vary across hippocampal areas and nAChR subtypes. Evidence exists for nicotine-facilitated LTP in CA1 of the hippocampus, but early studies did not distinguish high-affinity versus low-affinity nAChR involvement in these processes (Fujii, Ji, Morita, and Sumikawa, 1999; Ge and Dani, 2005). Recently, it has been established that nicotine-facilitated LTP occurs via mainly high-affinity nAChRs in area CA1 of the hippocampus in interneurons (Jia, Yamazaki, Nakauchi, Ito, and Sumikawa, 2010; Jia, Yamazaki, Nakauchi, and Sumikawa, 2009; Yamazaki, Jia, Hamaue, and Sumikawa, 2005) and pyramidal neurons (Fujii, Ji, and Sumikawa, 2000; Nakauchi, Brennan, Boulter, and Sumikawa, 2007; Nakauchi and Sumikawa, 2012; Rosato-Siri, Cattaneo, and Cherubini, 2006). Interestingly, nicotine may simultaneously enhance synaptic efficacy in Schaffer collateral (CA3–CA1 projections) neurons while inhibiting synaptic efficacy in entorhinal cortical projections to CA1 (Nakauchi et al., 2007), which may enhance or disrupt specific streams of information potentially altering what is learned. Additionally, nicotine potentiated synaptic transmission through high-affinity nAChRs while endogenous acetylcholine potentiated synaptic transmission through low-affinity receptors (Nakauchi and Sumikawa, 2012), indicating a dissociation between normal neural communication and drug-induced alterations. This dissociation could contribute to the ability of nicotine to alter neural signaling that leads to changes in learning and memory. In addition to nicotine facilitating LTP in the CA1 region of the hippocampus, nicotine can also potentiate synaptic signaling in the DG region of the hippocampus (Welsby, Rowan, and Anwyl, 2006; Welsby, Rowan, and Anwyl, 2009; Zhang, Tang, Pidoplichko, and Dani, 2010). Most likely, nicotine-facilitated synaptic plasticity in CA1 and DG of the hippocampus supports the enhancement of the various behaviors including spatial and contextual memory processing.
Nicotine can also induce LTP in the absence of tetanic stimulation. This nicotine-induced LTP occurs in CA1 pyramidal neurons (He, Deng, Chen, Zhu, and Yu, 2000; He, Deng, Zhu, Yu, and Chen, 2003; Wang, Chen, Zhu, and Chen, 2001) and DG granule neurons (Matsuyama and Matsumoto, 2003; Matsuyama, Matsumoto, Enomoto, and Nishizaki, 2000; Tang and Dani, 2009). In CA1, nicotine-induced LTP was found to be Ca2+ dependent but NMDAR-independent (He et al., 2000; He et al., 2003) and involve ERK1/2 signaling (Wang et al., 2001). While the canonical LTP pathway involves NMDAR-mediated Ca2+ influx, there is evidence that LTP can occur independently of NMDAR activation (Harris and Cotman, 1986), and alternative mechanisms such as nAChR activation may provide the necessary Ca2+ to induce synaptic potentiation. While the specific nAChR subtypes involved in nicotine-induced LTP were not directly tested in CA1, in the DG both low- and high-affinity nAChRs were critical for nicotine-induced LTP (Matsuyama and Matsumoto, 2003; Matsuyama et al., 2000). The ability of nicotine to directly induce synaptic plasticity may be one mechanism through which nicotine use can lead to the formation of strong maladaptive drug-associated memories. Thus, understanding how nicotine can both facilitate and directly induce synaptic plasticity may elucidate how nicotine use can result in long-lasting changes in behavior.
5.0 Acute Nicotine and Hippocampus-Dependent Learning
Nicotine, the main psychoactive component of tobacco products (USDHHS, 1988), is considered a cognitive enhancing drug (Rezvani and Levin, 2001). Though there is some debate on the cognitive effects as many of the studies that examined the effects of nicotine on cognitive function used abstinent smokers as subjects and thus observed procognitive effects could also be related to amelioration of withdrawal deficits (Heishman, Taylor, and Henningfield, 1994). Using a preclinical model it is possible to completely control for previous nicotine use and determine the extent to which initial nicotine use may affect learning. This approach has been used to examine the effects of acute nicotine on hippocampus-dependent and hippocampus-independent learning (fear conditioning and object recognition) in mice. With fear conditioning, a training session can involve two different types of learning: a tone-shock association (cued conditioning) that is hippocampus-independent and amygdala-dependent and a context-shock association (contextual conditioning) that is hippocampus- and amygdala-dependent (Fanselow, Kim, Yipp, and De Oca, 1994; Logue, Paylor, and Wehner, 1997; Phillips and LeDoux, 1992). A strength of this particular learning paradigm is that each subject concurrently learns two associations that involve unique networks of brain regions and thus if a drug has an effect on one type of learning and not the other, this result will inform on the behavioral and neural effects of the drug. Object recognition has two common variants: novel object recognition and spatial object recognition. For novel object recognition, subjects are placed in an arena devoid of spatial cues. The arena contains two different objects that the subjects can explore. On test day, one of the objects is replaced with a novel object. Because rodents will investigate a novel object more, subjects should explore the new object more if they learned and remembered the prior objects (Bevins and Besheer, 2006; Dere, Huston, and De Souza Silva, 2007; Ennaceur, 2010; Mathiasen and DiCamillo, 2010). The hippocampus is not necessary for this type of learning (Forwood, Winters, and Bussey, 2005; Winters, Forwood, Cowell, Saksida, and Bussey, 2004), although that is not to say the hippocampus is not recruited in a normal animal. Hippocampal lesions performed after extensive training impair performance in this task, indicating potential involvement in certain versions of this task (Broadbent, Gaskin, Squire, and Clark, 2009). Spatial object recognition is similar to novel object recognition in that there are two objects in an arena but in this case the arena contains spatial landmarks. On testing day, one of the objects is displaced to a new spatial location in the arena (Ennaceur, Neave, and Aggleton, 1997). If the subjects learned the spatial relationship between the objects and the environment, they should explore the object in the novel spatial location more. This type of learning is hippocampus-dependent (Barker and Warburton, 2011; Bussey, Duck, Muir, and Aggleton, 2000).
In multiple fear conditioning experiments, acute nicotine enhanced contextual but not cued conditioning (Davis, James, Siegel, and Gould, 2005; Davis, Porter, and Gould, 2006; Gould and Lommock, 2003; Gould and Wehner, 1999; Gulick and Gould, 2008; Portugal, Wilkinson, Kenney, Sullivan, and Gould, 2012a; Portugal, Wilkinson, Turner, Blendy, and Gould, 2012b). The lack of effect on cued conditioning was not due to ceiling effects, since increasing or decreasing the number of CS presentations did not reveal any effects of nicotine on cued fear conditioning (Gould, Feiro, and Moore, 2004). Additionally, altering the saliency of the context itself (by including or not including a highly salient, and therefore potentially overshadowing, auditory cue) does not change the effects of nicotine on contextual fear conditioning (Davis et al., 2006).
When a subject forms a contextual association with the shock, the subject must first learn the context and then form a context-shock association. As such, it has been shown that immediate early genes important for contextual learning are upregulated after exploration of a novel environment (Huff, Frank, Wright-Hardesty, Sprunger, Matus-Amat, Higgins, and Rudy, 2006; Leach, Poplawski, Kenney, Hoffman, Liebermann, Abel, and Gould, 2012; Ma, Jang, Guo, Kitabatake, Chang, Pow-Anpongkul, Flavell, Lu, Ming, and Song, 2009). Nicotine could enhance learning of the context itself, it could enhance the strength of the context-shock association, or it could enhance both processes. Normally during contextual fear conditioning, the contextual learning and the context-shock association occur during the same training session and so it is difficult to parse out which processes a drug is affecting. There is, however, a way around this limitation. The context pre-exposure facilitation effect allows for the separation of the contextual learning and the context-shock learning. Specifically, if subjects are placed in a conditioning chamber and immediately receive a shock presentation and are then removed, no contextual-shock association occurs presumably because the subject has not had sufficient time to learn the context. However, pre-exposure to the context the day before is sufficient to allow contextual conditioning (Fanselow, 1990). Using the context pre-exposure facilitory effect, nicotine administration on the context pre-exposure day was necessary to see the enhancement of contextual conditioning but nicotine administration at immediate shock was not sufficient (Kenney and Gould, 2008). This suggests that nicotine is specifically enhancing learning the context. Because of the selective involvement of the hippocampus in the contextual learning and the specificity of the nicotine effects of contextual versus cued conditioning, nicotine is likely either acting in the hippocampus or areas afferent or efferent of the hippocampus that are not critically involved in cued fear conditioning.
Additional studies further suggest that nicotine has specific affinity for hippocampal learning tasks. As discussed, cued fear conditioning does not normally depend on the hippocampus. However, if the parameters of cued fear conditioning are altered such that there is a temporal delay between the offset of the cue and the onset of the shock, the hippocampus is recruited. This type of learning, called trace fear conditioning is thought to engage the hippocampus because a memory trace of the cue must be maintained during the interval between cue offset and shock onset (McEchron, Bouwmeester, Tseng, Weiss, and Disterhoft, 1998). In support of this theory, Bangasser and colleagues (2006) showed that stimulus contiguity regulated hippocampal involvement in trace conditioning; such that in the absence of contiguity, the hippocampus was involved. Thus, the afferent and efferent connections of the hippocampus and the internal circuitry of the hippocampus (Rodriguez and Levy, 2001) may support a reverberating circuit necessary to maintain a memory trace (Bartesaghi et al., 1995; Witter, Groenewegen, Lopes da Silva, and Lohman, 1989). Since the hippocampus is recruited during trace conditioning, it would be predicted that while nicotine does not enhance standard cued fear conditioning, it would enhance trace fear conditioning; this is exactly what was seen (Davis and Gould, 2007b; Gould et al., 2004). This provides further support that a determining factor in the effects of nicotine on learning is the level of engagement of the hippocampus.
Just as nicotine enhances contextual learning, nicotine also enhances hippocampus-dependent spatial learning. A clear dissociation was seen for the effects of nicotine on spatial versus novel object recognition. While acute nicotine enhanced spatial object recognition, a deficit was seen in novel object recognition (Kenney, Adoff, Wilkinson, and Gould, 2011). This suggests that nicotine administration may shift cognitive processing to favor hippocampus-mediated processes. In addition to spatial object recognition, acute nicotine also enhances other spatial learning tasks as enhanced performance in the Morris water maze was seen in some studies (Abdulla, Bradbury, Calaminici, Lippiello, Wonnacott, Gray, and Sinden, 1996; Sharifzadeh, Tavasoli, Naghdi, Ghanbari, Amini, and Roghani, 2005; Socci, Sanberg, and Arendash, 1995), but not all studies (Attaway, Compton, and Turner, 1999; Bernal, Vicens, Carrasco, and Redolat, 1999); however, for the studies with the null results, one study tested only one dose of nicotine and the other tested two doses, leaving the possibility that other doses could be effective.
5.1 Localization of Nicotine Effects
Behavioral data suggests that nicotine is enhancing hippocampus-mediated processes but does not indicate if nicotine is directly acting in the hippocampus to enhance learning or altering afferent or efferent areas. Direct infusion of acute nicotine into the dorsal hippocampus enhanced contextual conditioning while infusion into cortex above or thalamus below had no effect on the learning (Davis, Kenney, and Gould, 2007; Kenney, Raybuck, and Gould, 2012b). Interestingly, infusion of nicotine into the ventral hippocampus disrupted contextual conditioning (Kenney et al., 2012b). As discussed earlier, the dorsal and ventral hippocampus should be viewed as functionally discrete areas, just as the core and shell of the nucleus accumbens are (Di Chiara, 2002; Kelley, 1999; Zahm, 1999; Zahm and Brog, 1992). The dorsal hippocampus may be more involved in contextual and spatial learning whereas the ventral hippocampus may be more involved in processing anxiety, stress, and emotions and learning associated with these processes (Fanselow and Dong, 2010). It is possible that during learning there is competition between the dorsal and ventral hippocampus for the type of learning and the behavioral response. Acute systemic nicotine administration, perhaps at specific doses, may favor processes mediated by the dorsal hippocampus but when nicotine is directly infused into the ventral hippocampus, enhancement of processes mediated by the ventral hippocampus, such as anxiety, could occur which would interfere with the expression of fear learning. To further understand the different effects of nicotine in the dorsal versus ventral hippocampus, it is necessary to examine the nAChRs involved.
Based on autoradiographic receptor binding studies, the predominant nAChRs in the hippocampus are α7 nAChRs (Dominguez del Toro et al., 1994) and those that bind agonist with high-affinity (e.g., α4β2 and α3β4) (Perry et al., 2002). A study that examined patterns of nAChR subunit mRNA expression found that the highest levels of nAChR transcripts were for α4 and β2 (Wada et al., 1989), which suggested that 4β2* nAChRs are the predominant high-affinity nAChR in the hippocampus. Traditionally, it was assumed that the 7 nAChR would be the dominant nAChR involved in hippocampus-dependent cognitive processes. The growth of this idea came from the discovery that patients with schizophrenia that had cognitive deficits such as impairments in sensory gating also had polymorphisms in the gene coding for the 7 subunit (Adler et al., 1998; Freedman et al., 1995; Leonard, Adams, Breese, Adler, Bickford, Byerley, Coon, Griffith, Miller, Myles-Worsley, Nagamoto, Rollins, Stevens, Waldo, and Freedman, 1996; Leonard et al., 2002). However, experiments in animal models have not supported a dominant role for 7 nAChRs in hippocampus-dependent learning. For contextual learning, dihydro-beta-erythoidine (DHβE), an antagonist for 4β2 nAChRs and to a lesser extent other high affinity nAChRs (Harvey and Luetje, 1996; Harvey, Maddox, and Luetje, 1996), did not disrupt learning but blocked the effects of nicotine on contextual learning (Davis and Gould, 2006; Davis et al., 2007). In contrast, the 7 nACh antagonist MLA neither disrupted contextual learning nor blocked the nicotine enhancement of the learning (Davis and Gould, 2006; Davis et al., 2007). Similar results were also seen in studies using genetically modified mice. Young β2 nAChR subunit knockout mice showed normal (Caldarone, Duman, and Picciotto, 2000; Davis and Gould, 2007b; Portugal, Kenney, and Gould, 2008) or modestly impaired contextual fear conditioning (Wehner, Keller, Keller, Picciotto, Paylor, Booker, Beaudet, Heinemann, and Balogh, 2004) but no enhancement of contextual conditioning by nicotine (Wehner et al., 2004). 7 knockout mice, on the other hand, showed both normal learning (Paylor, Nguyen, Crawley, Patrick, Beaudet, and Orr-Urtreger, 1998; Wehner et al., 2004) and nicotine-enhanced contextual learning (Wehner et al., 2004), but have shown deficits in sensory gating (Azzopardi, Typlt, Jenkins, and Schmid, 2013).
In another study, direct infusion of DHβE into the dorsal hippocampus prevented the enhancement of contextual learning by systemic administration of acute nicotine (Davis et al., 2007). This further demonstrated the necessity of the dorsal hippocampus for nicotine enhancement of learning and confirmed that 4β2* nAChRs in the dorsal hippocampus are mediating this effect of acute nicotine. Interestingly, infusion of MLA into the ventral hippocampus blocked the ability of nicotine to disrupt contextual learning (Kenney et al., 2012b). This suggests that 4β2* nAChRs in the dorsal hippocampus modulate contextual learning while 7 nAChRs in the ventral hippocampus may mediate nicotine effects that can act in opposition to contextual conditioning. Systemic nicotine would be expected to activate both receptors and yet enhancement of contextual learning is seen with acute nicotine. The reason for this may lie in the different properties of the 7 and 4β2 nAChRs. 7 nAChRs rapidly desensitize and have a higher threshold for activation compared to 4β2 nAChRs, which desensitize at a slower rate (Alkondon, Pereira, Almeida, Randall, and Albuquerque, 2000). Thus, systemic nicotine could conceivably preferentially activate 4β2 nAChRs at lower doses. At higher doses, 7 nAChRs may be activated and this may contribute to the inverted U-shaped doses response curve for the effects of nicotine on contextual conditioning (Gould and Higgins, 2003) and other behaviors (Picciotto, 2003). In addition, it has been shown that there is a greater density of 7 nAChRs in the ventral hippocampus (Mugnaini, Tessari, Tarter, Merlo Pich, Chiamulera, and Bunnemann, 2002), which may further contribute to the difference in the effects of nicotine infused into the dorsal hippocampus versus the ventral hippocampus and also highlights the functional and cellular differences between the dorsal and ventral hippocampus.
5.2 Nicotine and Hippocampal Cell Signaling
In order for nicotine to enhance hippocampus-dependent learning, cellular processes downstream from nAChRs must interact with cell signaling cascades involved in learning and memory. As discussed earlier, activation of NMDA receptors is critically involved in hippocampal plasticity and memory (Fanselow et al., 1994; Gould, McCarthy, and Keith, 2002; Huerta, Sun, Wilson, and Tonegawa, 2000; Morris, Anderson, Lynch, and Baudry, 1986; Nakazawa et al., 2002; Place, Lykken, Beer, Suh, McHugh, Tonegawa, Eichenbaum, and Sauvage, 2012; Tonegawa et al., 1996; Tsien et al., 1996) as NMDA receptor-mediated calcium influx activates cell signaling cascades involved in learning and memory (English and Sweatt, 1997; Platenik et al., 2000; Poser and Storm, 2001). NMDA receptors and nAChRs may mediate similar processes. While the nAChR antagonist mecamylamine failed to disrupt contextual fear conditioning when administered alone, a profound disruption of contextual fear conditioning was seen when paired with a subthreshold dose of the NMDA receptor antagonist MK801, (Gould and Lewis, 2005). In addition, nicotine infusion into the dorsal hippocampus reversed systemic MK801-induced deficits in contextual fear conditioning and systemic nicotine reversed the disruptive effects of direct infusion of the NMDA receptor antagonist DL-2-Amino-5-phosphonovaleric acid (APV) into the dorsal hippocampus. These effects were mediated by 4β2*, but not 7, nAChRs as DHβE but not MLA blocked the ability of nicotine to reverse the deficits (Andre, Leach, and Gould, 2011). Thus, it is possible that the activation of nAChR leads to a greater Ca2+ influx or internal release, which then modulates learning-related cell signaling cascades. In support, Stitzel and colleagues (2004) showed that nicotine evoked Ca2+ influx through 4β2 nAChRs and this led to a release of Ca2+ from internal Ca2+ stores. Higher intercellular Ca2+ levels associated with nicotine administration could alter the activation of cell signaling cascades involved in learning and memory.
As discussed earlier, PKA and ERK are involved in hippocampus-dependent learning (Abel and Nguyen, 2008; Abel et al., 1997; Atkins et al., 1998; Bernabeu et al., 1997). Nicotine may enhance hippocampus-dependent learning through greater activation of PKA and ERK (figure 2) as administration of a PKA inhibitor (Wilkinson, Yildirim, Poole, Leach, and Gould, 2012) and an ERK inhibitor (Raybuck and Gould, 2007) at doses subthreshold for altering learning blocked the enhancement of learning by nicotine. This suggests that during learning nicotine administration increases the active pool of PKA and ERK and if this increase is blocked, no enhancement of learning is seen. Because long-term memory is associated with changes in gene transcription linked to PKA and ERK activation (Abel and Nguyen, 2008; Impey et al., 1998a; Impey et al., 1998b; Silva et al., 1998), the effects of nicotine on the pattern of genes activated during learning was investigated. It was found that nicotine paired with learning led to an increase in expression of Jnk1 in the hippocampus (Kenney, Florian, Portugal, Abel, and Gould, 2010) and was associated with increased CREB phosphorylation at the Jnk1 promoter region in the hippocampus (Kenney, Poole, Adoff, Logue, and Gould, 2012a). Both the increased expression of Jnk1 and the increased CREB phosphorylation at the Jnk1 promoter were mediated through nicotine effects at β2-containing nAChRs (most likely 4β2* nAChRs) as these effects were absent in β2 KO mice (Kenney et al., 2010; Kenney et al., 2012a). Interestingly, the increase is Jnk1 expression was not seen with nicotine administration in the absence of learning or learning in the absence of nicotine. In addition, direct infusion of a pan-JNK inhibitor into the dorsal hippocampus during consolidation blocked the nicotine enhancement of learning whereas infusion of the same dose of the inhibitor prior to training or prior to recall in both nicotine-treated and nicotine naïve animals had no effect on learning. These results suggest that nicotine recruits cell signaling cascades that may not normally be involved in learning and memory, and activation of these cascades results in a stronger hippocampus-dependent memory. The mechanism through which the nicotine-associated JNK activation results in enhanced learning is unknown but JNK1 can phosphorylate microtubule-associated proteins (Bjorkblom, Ostman, Hongisto, Komarovski, Filen, Nyman, Kallunki, Courtney, and Coffey, 2005; Chang, Jones, Ellisman, Goldstein, and Karin, 2003), which could stabilize synapses, and JNK1 can activate transcription factors, such as the JUN family, ATF-2, and ELK-1 (Bogoyevitch and Kobe, 2006; Gupta, Barrett, Whitmarsh, Cavanagh, Sluss, Derijard, and Davis, 1996), that could modulate synaptic plasticity (Li, Li, Yu, Chen, Sabapathy, and Ruan, 2007; Sananbenesi, Fischer, Schrick, Spiess, and Radulovic, 2002; Strekalova, Zorner, Zacher, Sadovska, Herdegen, and Gass, 2003). The ability of nicotine to alter ongoing cell signaling cascades involved in learning and recruit additional cell signaling cascades may be one reason why nicotine administration is associated with the formation of strong drug-context memory that can contribute to drug seeking behavior (Portugal and Gould, 2009; Walters, Cleck, Kuo, and Blendy, 2005; Wilkinson and Bevins, 2008).
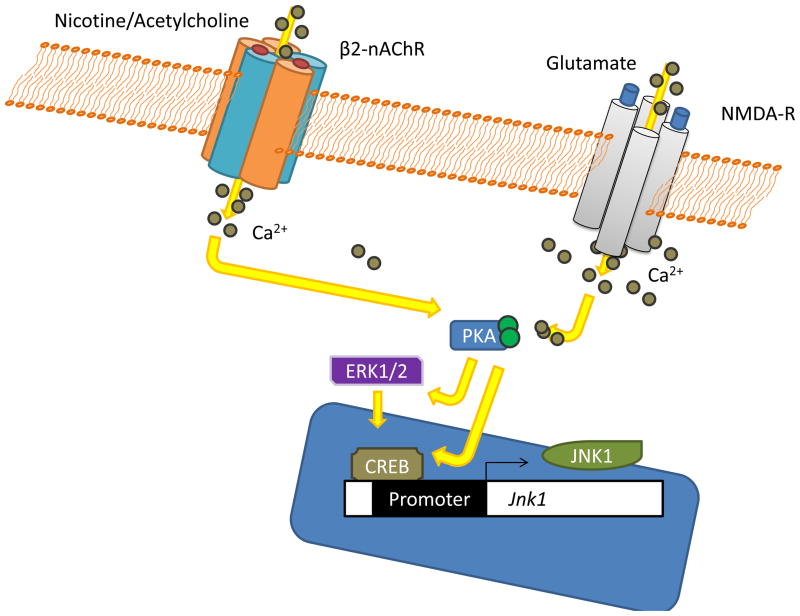
Cell signaling cascade important for nicotine’s acute effects on hippocampus-dependent learning. Nicotine activates nAChRs which may lead to an increase in intracellular calcium or may provide the necessary depolarization to allow NMDA-receptor mediated calcium influx. Calcium leads to the activation of PKA and ERK, which then activate CREB. CREB activation stimulates Jnk1 transcription, and JNK activation is critical for nicotine-enhanced learning.
6.0 Chronic and Withdrawal from Chronic Nicotine and Hippocampus-Dependent Learning
Addiction is a complex disorder as the effects of drugs of abuse vary across substances. Thus, it is not too surprising that abstinence symptoms (also known as withdrawal) differ with different drugs of abuse. For nicotine, two of the most common withdrawal symptoms are changes in affect and changes in cognition (Bell, Taylor, Singleton, Henningfield, and Heishman, 1999; Hughes, 2007; Hughes, Gust, Skoog, Keenan, and Fenwick, 1991; Kleinman, Vaughn, and Christ, 1973; Snyder, Davis, and Henningfield, 1989). In fact, changes in cognition during periods of abstinence from smoking predicts relapse (Patterson, Jepson, Loughead, Perkins, Strasser, Siegel, Frey, Gur, and Lerman, 2010; Rukstalis, Jepson, Patterson, and Lerman, 2005). Hence, understanding the behavioral and biological substrates for the effects of nicotine withdrawal on cognition should advance treatment of nicotine addiction, yet this area of research is relatively new. Studies in humans have shown that abstinence from smoking is associated with difficulty in concentration (Hendricks, Ditre, Drobes, and Brandon, 2006; Hughes et al., 1991; Hughes, Higgins, and Bickel, 1994), impairments in attention (Hughes, Keenan, and Yellin, 1989; Jacobsen, Krystal, Mencl, Westerveld, Frost, and Pugh, 2005), deficits in learning and memory (Jacobsen et al., 2005; Jacobsen, Mencl, Constable, Westerveld, and Pugh, 2007; Mendrek, Monterosso, Simon, Jarvik, Brody, Olmstead, Domier, Cohen, Ernst, and London, 2006; Merritt, Cobb, Moissinac, and Hirshman, 2010; Snyder et al., 1989), and disrupted cortical plasticity (Grundey, Thirugnanasambandam, Kaminsky, Drees, Skwirba, Lang, Paulus, and Nitsche, 2012). However, few studies have used the strengths of rodent models to investigate the neural substrates that mediate the effects of nicotine withdrawal on cognition. An exception to this has been studies of the effects of nicotine withdrawal on learning in mice.
As discussed earlier, acute nicotine enhances hippocampus-dependent learning (Gould and Higgins, 2003; Gould and Wehner, 1999). However, this effect disappears with chronic nicotine treatment, indicating tolerance has developed, and when chronic nicotine treatment is ended, hippocampus-dependent learning is disrupted (Davis et al., 2005). This suggests that with chronic nicotine use, adaptations occur that alter brain function resulting in tolerance and then deficits in learning are uncovered when nicotine administration ceases. In mice, it has been consistently shown that nicotine withdrawal disrupts hippocampus-dependent but not hippocampus-independent learning (Davis et al., 2005; Kenney et al., 2011; Portugal et al., 2012a). The selectivity of the withdrawal effects on hippocampus-dependent learning demonstrates that the withdrawal deficits are not due to nonspecific effects (e.g., changes in locomotion or malaise) that would impact multiple behaviors and also suggests that chronic nicotine and subsequent withdrawal are either directly altering the hippocampus or afferent and/or efferent areas. In order to fully understand the effects of nicotine withdrawal on cognition, it is important to identify the neural substrates involved in the withdrawal-related deficits in learning, whether the same mechanisms involved in the acute effects of nicotine on learning are involved in the withdrawal symptoms, and what aspects of learning processes are altered during nicotine withdrawal. Answering these questions will advance our understanding of nicotine addiction and aid in developing more effective treatments.
Learning consists of multiple stages (acquisition, consolidation, recall) and nicotine withdrawal could potentially affect any or all of these stages. A nicotine withdrawal-associated disruption of acquisition and/or consolidation would mean that smokers would have difficulties with new learning during periods of abstinence but may not have difficulty in the recall of older memories. Whereas a deficit in memory retrieval would produce a much larger deficit as both new and old memories would be disrupted or at least inaccessible. Because mice will develop a preference for contexts associated with nicotine administration (Risinger and Oakes, 1995), they can be used to investigate these maladaptive drug-context associations. The drug-context learning is hippocampus-dependent (Meyers, Zavala, and Neisewander, 2003; Sakurai, Yu, and Tan, 2007; Shen, Meredith, and Napier, 2006) (but see (McDonald, Yim, Lehmann, Sparks, Zelinski, Sutherland, and Hong, 2010)) and may contribute to future drug seeking behavior (Fuchs, Evans, Ledford, Parker, Case, Mehta, and See, 2005). To test if nicotine withdrawal altered the learning or the recall of contextual memories, mice that developed a conditioned place preference for a nicotine-associated context were administered chronic nicotine, withdrawn from treatment, tested for the old nicotine-context memories, and then trained in new hippocampus-dependent contextual learning (Portugal and Gould, 2009). Mice withdrawn from chronic nicotine showed strong preference for the nicotine-paired context but had deficits in acquisition of new contextual learning. Thus, the recall of old drug-context memories remained intact during withdrawal while new hippocampus-dependent learning was disrupted. This finding suggests how the effects of nicotine on hippocampus-dependent learning may contribute to maintenance of nicotine addiction. Acute or initial nicotine use may facilitate the development of strong drug-context associations that contribute to drug seeking behavior. During periods of abstinence, these drug memories remain strong while acquisition of new and perhaps adaptive memories is disrupted. Frustration with cognitive difficulties and an inability to learn adaptive coping strategies combined with the presence of strong drug reward-related memories could result in relapse. In support, Tiffany (1999) proposed that during abstinence drug-associated memories dominate and usurp cognitive capacity. Thus, treating these changes in cognition could facilitate abstinence; however, effective therapeutic development requires understanding of the neurobiological basis for the symptoms.
In order to understand how chronic nicotine and subsequent withdrawal change behavior and brain function, the specific neural areas altered must be identified. While systemic behavioral pharmacological studies have suggested that nicotine is working in the hippocampal system (Andre, Gulick, Portugal, and Gould, 2008; Davis et al., 2005; Kenney et al., 2011; Portugal and Gould, 2009; Portugal et al., 2012b; Raybuck and Gould, 2009), direct drug infusion studies specifically identified were nicotine acts to produce withdrawal-related changes in learning. Withdrawal from chronic infusion of nicotine into the dorsal hippocampus disrupted learning whereas withdrawal from chronic infusion into the cortical area above the hippocampus or the thalamic region directly below the hippocampus did not disrupt learning (Davis and Gould, 2009). These results suggest that chronic nicotine specifically changes dorsal hippocampal function. In addition, it was demonstrated that nicotine withdrawal deficits in hippocampus-dependent learning could be precipitated by directly infusing a nicotinic receptor antagonist, DHβE, into the dorsal hippocampus of mice treated chronically with systemic nicotine (Davis and Gould, 2009). Together, these studies demonstrate that the effects of chronic nicotine in the dorsal hippocampus are both necessary and sufficient to produce withdrawal-associated deficits in learning, and because DHβE is an antagonist for high-affinity nAChRs receptors such as the 4β2 nAChR (Harvey and Luetje, 1996; Harvey et al., 1996), they also suggest that nicotine is acting on 4β2* nAChRs in the hippocampus to produce the changes necessary for withdrawal disruption of learning. The evidence for 4β2* nAChR involvement was further strengthened by studies that demonstrated that nicotine withdrawal deficits did not develop in mice with the β2 nAChR subunit knocked out but did develop in wild-type mice and mice with the 7 nAChR subunit knocked out (Davis and Gould, 2009; Portugal et al., 2008; Raybuck and Gould, 2009). In addition, the 7 nAChR antagonist MLA did not precipitate withdrawal deficits in hippocampus-dependent learning in mice treated with chronic nicotine, contrary to the effects seen with DHβE (Davis and Gould, 2009). Thus, chronic nicotine alters signaling through dorsal hippocampal high-affinity nAChRs, most likely 4β2* nAChRs.
Identifying the brain region and receptor subtypes involved in the effects of chronic nicotine and subsequent withdrawal on behavior are important initial steps for developing specific therapeutic targets for nicotine addiction but it is also necessary to identify the mechanism by which the brain is changed. As discussed previously, nicotine is an agonist yet with chronic treatment, it will desensitize specific subtypes of nAChRs (Chavez-Noriega et al., 1997; Olale et al., 1997) while other receptor subtypes are not changed (Mao et al., 2008). Along with the desensitization, upregulation of nAChRs is seen and the degree and duration of upregulation varies by nAChR subtype (Barrantes et al., 1995; Marks et al., 1985; Rogers and Wonnacott, 1995). 4β2 nAChRs, a high-affinity nAChR and the subtype most likely involved in nicotine withdrawal effects on hippocampus-dependent learning (Davis and Gould, 2009; Portugal et al., 2008; Raybuck and Gould, 2009), show strong upregulation that lasts longer than other nAChR subtypes (Marks et al., 1985). Smokers during early abstinence show higher levels of β2-containing nAChRs (Staley, Krishnan-Sarin, Cosgrove, Krantzler, Frohlich, Perry, Dubin, Estok, Brenner, Baldwin, Tamagnan, Seibyl, Jatlow, Picciotto, London, O’Malley, and van Dyck, 2006). Thus, maintained functional upregulation of nAChRs is one potential mechanism responsible for observed withdrawal symptoms (Dani and Heinemann, 1996). We specifically propose that chronic nicotine desensitizes, but also upregulates, high-affinity 4β2 nAChRs in the dorsal hippocampus. When chronic treatment ceases, desensitized nAChRs regain sensitivity while 4β2 nAChRs remain upregulated (figure 3). This would result in a sensitized nAChR system. Several predictions emerge from this model. First, the duration of withdrawal deficits in learning should match the duration of 4β2 nAChR upregulation. Second, conditions that do not result in withdrawal deficits in learning should not produce upregulation of nAChRs. Third, drugs that reduce 4β2 nAChR function, such as partial agonists, should reduce withdrawal-related cognitive deficits.
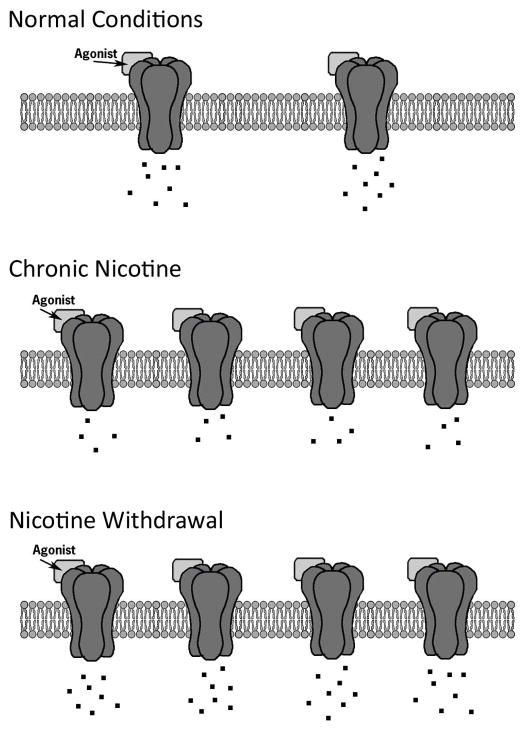
Model of potential withdrawal-related changes in nAChR function. The top schematic represents agonist stimulated ion influx at baseline (i.e., prior to chronic nicotine exposure). With chronic nicotine exposure, receptors upregulate and desensitize (middle), which leaves the overall activity and ion influx approximately equivalent to baseline levels. During abstinence, upregulated receptors may regain sensitivity to agonists (bottom), leaving the system with a gain in ion influx and potentially in a hyperfunctional state. This change may contribute to withdrawal deficits in learning. Filled square represent Na+ or Ca2+.
In general, nicotine withdrawal symptoms abate over time (Hughes, 2007), which suggests that the underlying changes in brain function should also dissipate in a similar time frame. In mice, nicotine withdrawal deficits in hippocampus-dependent learning lasted 4 days and by the 5th day, learning was similar to controls (Gould et al., 2012). Interestingly, the duration of high-affinity nAChR upregulation in the hippocampus closely paralleled the duration of behavioral deficits. This pattern of upregulation was not seen in cortex or cerebellum. The significance of this is that the nicotine withdrawal-associated deficits in learning were specifically related to nicotine effects in the hippocampus and suggest that the changes in high-affinity nAChR upregulation in the hippocampus are an important contributing factor. Future studies are needed that examine if similar changes in nAChR upregulation are related to other withdrawal symptoms.
7.0 Genetic Influences
The studies discussed so far were conducted in C57BL/6 mice but genotype affects phenotype (Logue, Owen, Rasmussen, and Wehner, 1997; Owen, Logue, Rasmussen, and Wehner, 1997; Tarantino and Eisener-Dorman, 2012; Tarantino, Gould, Druhan, and Bucan, 2000). In an examination of the effects of acute, chronic, and withdrawal from chronic nicotine on hippocampus-dependent learning in 8 different inbred strains of mice, it was found that genetic background differentially affected the acute and withdrawal effects of nicotine on hippocampus dependent learning (Portugal et al., 2012a). For example, C57BL/6 mice were sensitive to the effect of both acute nicotine and withdrawal from chronic nicotine, whereas the 129/SvEv strain showed sensitivity to the acute effects but not to the withdrawal effects (Portugal et al., 2012a). The results of this study demonstrated that genotype affects withdrawal symptoms, which suggests that in smokers, withdrawal symptoms will vary based on genetic background and thus effective treatments may one day be tailored to genotype. In addition, because the acute effects of nicotine on learning across strains were not always predictive of the effects of nicotine withdrawal on learning, these results also suggest that the genetic factors underlying the acute effects of nicotine on learning may differ from the genetic factors underlying the withdrawal deficits in learning (Portugal et al., 2012a). In other words, if the same genes contribute to the effects of acute nicotine and nicotine withdrawal on learning, then there should have been a strong correlation between the acute and withdrawal effects across strains; this was not seen.
If hippocampal nAChR upregulation is related to withdrawal deficits in hippocampus-dependent learning, as previous results suggest (Gould et al., 2012; Portugal et al., 2012a), then strains of mice that do not do not show the withdrawal deficits in learning should also not show nAChR upregulation. To examine this, C57Bl/6N mice, 129/SvEv mice, and the F1 cross B6129SF1 were examined for withdrawal deficits in hippocampus-dependent and hippocampus-independent learning and for changes in dorsal and ventral hippocampal nAChR upregulation (Wilkinson, Turner, Blendy, and Gould, 2012). No group showed withdrawal-related deficits in hippocampus-independent learning, as expected, and only the C57BL/6N mice showed withdrawal-related deficits in hippocampus-dependent learning. The C57BL/6N mice were also the only group that showed upregulation of high-affinity nAChRs in the dorsal hippocampus. No change in ventral hippocampal nAChR upregulation was seen except for in the B6129SF1 mice, and this did not correlate with any learning-related changes. These results again demonstrate a relationship between nAChR upregulation in the dorsal hippocampus and the expression of withdrawal-related deficits in hippocampus-dependent learning while further highlighting dorsal and ventral hippocampal differences.
An increase in active nAChRs during nicotine withdrawal could lead to increased sensitivity for activation of associated neural circuitry by nicotine and acetylcholine. In support, nicotine abstinence produces cognitive deficits associated with greater activation of frontal lobe function, including cingulate cortex (Jacobsen et al., 2007). In addition, withdrawal from a 1 week nicotine treatment produced a persistent increase in CA1 pyramidal cell excitability (Penton, Quick, and Lester, 2011). If sustained upregulation of high-affinity nAChRs results in hyperactive neural systems and this contributes to cognitive and learning withdrawal deficits, then a drug that reduces nAChR function should be efficacious in treating these symptoms.
8.0 Therapeutics
Nicotine addiction is a difficult disease to treat. Prolonged abstinence is rare and most therapeutics are only mildly successful. Currently, varenicline is the most effective drug treatment (Hudmon, Corelli, and Prokhorov, 2010). Varenicline is a partial agonist for 4β2 nAChRs and a full agonist for 7 nAChRs but has a >5000 affinity for 4β2 nAChRs compared to 7 nAChRs (Mihalak, Carroll, and Luetje, 2006). In rodent models, varenicline reduced nicotine self-administration and was less efficacious in stimulating dopamine release than nicotine (Rollema, Chambers, Coe, Glowa, Hurst, Lebel, Lu, Mansbach, Mather, Rovetti, Sands, Schaeffer, Schulz, Tingley, and Williams, 2007). In smokers, 52 week abstinence levels were at 22–23% for those treated with varenicline compared to 8–10% for those given a placebo (Gonzales, Rennard, Nides, Oncken, Azoulay, Billing, Watsky, Gong, Williams, and Reeves, 2006; Jorenby, Hays, Rigotti, Azoulay, Watsky, Williams, Billing, Gong, and Reeves, 2006). These results demonstrate therapeutic validity for varenicline but many factors can contribute to abstinence and it was unclear if varenicline would ameliorate cognitive withdrawal symptoms. Because withdrawal deficits in learning involve 4β2* nAChRs (Davis and Gould, 2009; Gould et al., 2012; Portugal et al., 2008; Raybuck and Gould, 2009) and may be related to hypersensitivity of nAChRs (Dani and Heinemann, 1996; Gould et al., 2012), there was reason to believe that the partial 4β2 nAChR agonist varenicline could ameliorate nicotine withdrawal-related deficits in learning. This was confirmed in a study examining nicotine withdrawal-related learning deficits in mice (Raybuck, Portugal, Lerman, and Gould, 2008). Studies in smokers further demonstrated that varenicline was effective in treating cognitive withdrawal symptoms (Loughead, Ray, Wileyto, Ruparel, Sanborn, Siegel, Gur, and Lerman, 2010; Patterson, Jepson, Strasser, Loughead, Perkins, Gur, Frey, Siegel, and Lerman, 2009; Rhodes, Hawk, Ashare, Schlienz, and Mahoney, 2012). The findings of these studies demonstrate that varenicline has efficacy in treating cognitive withdrawal symptoms while also providing support for the hypothesis that these nicotine withdrawal deficits may be related to hypersensitivity of nAChRs. In fact, results from a recent study suggest that during withdrawal there is increased sensitivity to the acute effects of nicotine on learning (Wilkinson and Gould, 2013). Thus, as a partial 4β2 nAChR agonist, varenicline may bind to those receptors preventing endogenous and exogenous ligands from fully activating them (Papke, Trocme-Thibierge, Guendisch, Al Rubaiy, and Bloom, 2011).
Not all evidence fully supports the argument that nicotine withdrawal deficits are associated with an increased pool of active nAChRs and thus a sensitization of the hippocampal nACh system. Nicotine agonists and drugs that enhance cholinergic signaling also ameliorate nicotine withdrawal deficits, which could suggest that during withdrawal the nicotinic system is hypofunctional. Specifically, nicotine and galantamine, an acetylcholinesterase inhibitor and an allosteric positive modulator of nAChRs (Harvey, 1995; Samochocki, Zerlin, Jostock, Groot Kormelink, Luyten, Albuquerque, and Maelicke, 2000), both ameliorate nicotine withdrawal deficits (Davis et al., 2005; Wilkinson and Gould, 2011). It may be the case, though, that during nicotine withdrawal upregulated active nAChRs are rapidly desensitized by nicotinic (full) agonists and higher levels of acetylcholine and this may contribute to the therapeutic actions of associated drugs. Clearly, this issue requires further research as resolving this may also improve pharmacotherapeutic development.
In addition to withdrawal altering cholinergic processes, it is possible that other neurotransmitter systems are affected. While nicotinic receptors are found at both pre and postsynaptic locations in the hippocampus (Alkondon et al., 1996; Araujo et al., 1988; Clarke and Reuben, 1996; Fabian-Fine et al., 2001; Gray et al., 1996; Radcliffe et al., 1999; Wilkie et al., 1996; Zarei et al., 1999), presynaptic locations could modulate the release of neurotransmitters associated with learning-related cell signaling cascades. Nicotine has been shown to evoke the release of acetylcholine, dopamine, norepinephrine, serotonin, glutamate, and GABA in the hippocampus (Alkondon, Braga, Pereira, Maelicke, and Albuquerque, 2000; Arqueros, Naquira, and Zunino, 1978; Barik and Wonnacott, 2006; Marchi, Risso, Viola, Cavazzani, and Raiteri, 2002; Shearman et al., 2005; Tani, Saito, Imoto, and Ohno, 1998; Tucci, Genn, and File, 2003). It is possible that the effects of nicotine withdrawal on hippocampus-dependent learning are mediated through presynaptic modulation of neurotransmitter release. For instance, atomoxetine, a norepinephrine-reuptake inhibitor (Kratochvil, Vaughan, Harrington, and Burke, 2003), and bupropion, a dopamine and norepinephrine reuptake inhibitor (Li, Perry, and Wong, 2002), reduced nicotine withdrawal-associated deficits in learning (Davis and Gould, 2007a; Portugal and Gould, 2007). These results could be interpreted as nicotine withdrawal affecting learning via modulation of noradrenergic signaling, however, bupropion is also a nAChR antagonist (Fryer and Lukas, 1999; Slemmer, Martin, and Damaj, 2000) and atomoxetine can indirectly modulate acetylcholine levels (Tzavara, Bymaster, Overshiner, Davis, Perry, Wolff, McKinzie, Witkin, and Nomikos, 2006); leaving open the modulation of cholinergic signaling as the primary mechanism of action. Therefore, this issue remains unresolved.
9.0 Other Cognitive Processes
This section of the review has largely focused on the effects of nicotine on hippocampus-dependent learning but other rodent studies have examined additional cognitive measures. In rats, both spontaneous withdrawal and precipitated withdrawal disrupted sustained attention in the 5-choice serial reaction time task (Shoaib and Bizarro, 2005). These deficits may involve high-affinity nAChRs such as 4β2* nAChRs because the high-affinity nAChR antagonist DHβE, but not the 7 nAChR antagonist MLA, precipitated withdrawal deficits. Another study also found that nicotine withdrawal was associated with altered performance of the 5-choice serial reaction time task; rats showed increased omitted responses and decreased correct responses (Semenova, Stolerman, and Markou, 2007). However, as discussed earlier, not all types of learning are disrupted by nicotine withdrawal as cued fear conditioning, an amygdala-dependent hippocampus-independent form of learning (Davis et al., 2005), novel object recognition (Kenney et al., 2011), radial arm maze performance (Levin, Briggs, Christopher, and Rose, 1992; 1993) and an appetitive operant task (Leach, Cordero, and Gould, 2013) were not disrupted by nicotine withdrawal. Thus, nicotine withdrawal has specific effects on particular cognitive processes. Further examination of other cognitive tasks may help identify what processes are specifically disrupted by nicotine withdrawal.
10.0 Adolescence, Nicotine, and Learning
The studies reviewed so far have largely focused on adult subjects but it is well established that adolescence is a period of increased experimentation with drugs of abuse, including tobacco products. According to the Monitoring the Future study, conducted at the University of Michigan in Ann Arbor, around 20% of high school seniors have used cigarettes in the last 30 days (Johnston, O’Malley, Bachman, and Schulenberg, 2012). Furthermore, the National Household Survey on Drug Abuse (NHSDA) reported in 2000 that 1.6 million adolescents ages 12–17 initiated tobacco use and 747,000 became daily smokers (USDHHS, 2003). In addition, earlier initiation of smoking predicted less success in quitting; compared to smokers that initiated smoking at age 13 or younger, smokers that initiated smoking between 14–16 and 17 or older were 1.6 and 2.0 (respectively) times more likely to quit (Breslau and Peterson, 1996). As stated previously, studies that examine the effects of nicotine withdrawal or abstinence from smoking on brain and behavior often use adult subjects; however, the assumption cannot be made that the adult brain is the same as the adolescent brain.
Increasing evidence shows that the adolescent brain is indeed different than the adult brain (Blakemore and Robbins, 2012; Casey, Jones, and Hare, 2008; Laviola, Adriani, Terranova, and Gerra, 2000; Spear, 2010). Not surprisingly, nicotine has different effects on adolescent brain function and behavior than in adults. Adolescent rodents were more sensitive to the rewarding and reinforcing effects of nicotine than adults (Kota, Robinson, and Imad Damaj, 2009; Shram and Le, 2010; Torres, Tejeda, Natividad, and O’Dell, 2008). Age-related differences also emerge for the effects of nicotine on hippocampus-dependent learning. Early adolescent mice were more sensitive to the effects of acute nicotine on hippocampus-dependent learning, showing nicotine-enhanced learning over a broader range of doses compared to older mice (Portugal et al., 2012b). Interestingly, this pattern was reversed for withdrawal from chronic nicotine. The younger mice were less affected while older mice showed nicotine withdrawal deficits in hippocampus-dependent learning across more doses of chronic nicotine. The reason for this age-related difference in sensitivity to nicotine withdrawal on learning is not clear but it was found that the younger mice not expressing withdrawal deficits in hippocampus-dependent learning also failed to show significant upregulation of high-affinity nAChRs in the hippocampus; whereas the adult mice that had withdrawal deficits in hippocampus-dependent learning also had upregulated hippocampal nAChRs. This finding further supports the idea that upregulation of high-affinity nAChRs is critically involved in withdrawal deficits in hippocampus-dependent learning.
Because the adolescent brain is still developing (Casey, Tottenham, Liston, and Durston, 2005), exposure to psychoactive drugs may have long-term consequences. Adolescent nicotine exposure is associated with adult depression (Iniguez, Warren, Parise, Alcantara, Schuh, Maffeo, Manojlovic, and Bolanos-Guzman, 2009) and cognitive deficits (Counotte, Spijker, Van de Burgwal, Hogenboom, Schoffelmeer, De Vries, Smit, and Pattij, 2009). It is, however, difficult to differentiate in humans whether smoking was the cause of the deficits or if smoking was initiated in an attempt to self-medicate pre-existing conditions. Both depression (Bremner, Narayan, Anderson, Staib, Miller, and Charney, 2000; Davidson, Pizzagalli, Nitschke, and Putnam, 2002; Duman, Heninger, and Nestler, 1997; Duman and Voleti, 2012; Nestler, Barrot, DiLeone, Eisch, Gold, and Monteggia, 2002) and cognition (Penfield and Milner, 1958) involve the hippocampus. This suggests that the adolescent hippocampus may be sensitive to effects of nicotine exposure and that this sensitivity may lead to long-term behavioral deficits. To test if adolescent nicotine exposure altered adult hippocampus-dependent learning, early-adolescent, late-adolescent, and adult mice were administered chronic nicotine for 12 days and then trained and tested for hippocampus-dependent and hippocampus-independent learning 30 days after the cessation of nicotine treatment (Portugal et al., 2012b). Mice treated with nicotine during early or late adolescence showed deficits in hippocampus-dependent learning in adulthood in contrast to the group treated with nicotine during adulthood. In addition, the younger the mice, the more sensitive they were to the effects of chronic nicotine exposure on future adult hippocampus-dependent learning. No groups displayed deficits in hippocampus-independent learning demonstrating that this was not a global cognitive deficit. In addition to changes in learning, other cognitive process may also be affected as adolescent nicotine exposure has also been shown to alter adult performance in the 5-choice serial reaction time task (Counotte et al., 2009; Schneider, Bizarro, Asherson, and Stolerman, 2012).
Increasing evidence suggests that adolescent nicotine exposure may be altering cell-signaling processes involved in gene transcription and translation. Signaling through the adenylyl cyclase/cAMP pathway can produce short-lasting changes that localize to areas of activation or can lead to long-lasting neural changes through the activation of gene transcription factors such as CREB (Borrelli, Montmayeur, Foulkes, and Sassone-Corsi, 1992; Poser and Storm, 2001; Tamai, Monaco, Nantel, Zazopoulos, and Sassone-Corsi, 1997). Adolescent rats treated with chronic nicotine had increased adenylyl cyclase activity in multiple brain regions (Abreu-Villaca, Seidler, and Slotkin, 2003; Xu, Seidler, Cousins, Slikker, and Slotkin, 2002). Increased adenylyl cyclase could lead to alterations in gene transcription and thereby impact development. To investigate this, the gene transcription factor CREB was examined in the hippocampus, cortex, and cerebellum 24 hours after cessation of chronic nicotine treatment in a set of early-adolescent and adult mice (Portugal et al., 2012b). Total CREB was increased in the cortex and decreased in the hippocampus of adolescent mice treated with chronic nicotine compared to saline-treated adolescent mice and adult mice treated with nicotine or saline. In the cerebellum, total CREB was decreased in mice treated with chronic nicotine for both age groups. CREB is involved in the development of the nervous system and learning and memory (for review see Lonze and Ginty, 2002). Therefore, changes in CREB during development could alter naturally occurring patterns of gene expression, impacting the development of the cortex and the hippocampus. In support, age-dependent changes in hippocampal gene expression were found in adolescent rats treated with chronic nicotine (Polesskaya, Fryxell, Merchant, Locklear, Ker, McDonald, Eppolito, Smith, Wheeler, and Smith, 2007), several of the identified genes are related to development and plasticity. Furthermore, adolescent nicotine exposure produced cell loss and decreased neurite outgrowth in the hippocampus and other areas (Abreu-Villaca, Seidler, Tate, and Slotkin, 2003); processes that can be mediated by changes in gene expression (Estus, Zaks, Freeman, Gruda, Bravo, and Johnson, 1994; Naeve, Ramakrishnan, Kramer, Hevroni, Citri, and Theill, 1997; Yamauchi, Miyamoto, Murabe, Fujiwara, Sanbe, Fujita, Murase, and Tanoue, 2007). Changes in gene expression could alter how the brain is wired and change adult function of cell signaling systems. This is seen for the cholinergic system; adolescent nicotine administration was associated with a decreased response of nAChRs to nicotine administration in adulthood (Slotkin, Bodwell, Ryde, and Seidler, 2008). Thus, the long-term neural and behavioral effects of adolescent nicotine exposure could produce compromised cognitive processes that contribute to adult mental illnesses and susceptibility to addiction. DeBry and Tiffany (2008) hypothesize that adolescent nicotine exposure has a toxic effect on cognitive development resulting in deficits in executive function that contribute to the development of addiction through disregulation of limbic and reward processes. Nicotine-related changes in CREB during adolescence could be one factor contributing to deficits in executive function. Clearly, the mechanisms through which adolescent nicotine exposure produce long-lasting changes in adults is an important area for further research.
11.0 Conclusion
Nicotine has direct effects in the hippocampus that alter learning and memory and these changes in cognitive function may impact susceptibility to developing and maintaining nicotine addiction. Acute administration of nicotine enhances hippocampus-dependent learning. This is mediated by processes in the dorsal hippocampus and involves activation of cell signaling cascades normally and those not normally involved in learning. The ability of nicotine to enhance learning processes could facilitate the development of maladaptive drug-context associations that can later evoke cravings and lead to drug seeking behavior. Interestingly, the studies reviewed here also demonstrated that the effects of nicotine in the hippocampus differ across the dorsal and ventral poles. This finding is part of a growing body of data that transcends research areas and demonstrates that the dorsal hippocampus and ventral hippocampus are strikingly different.
With chronic nicotine treatment tolerance develops, and cessation of treatment results in deficits in hippocampus-dependent learning. Deficits in learning and other cognitive processes are major symptoms of nicotine withdrawal and are strongly associated with relapse. Work in mice has identified an association between nAChR upregulation in the hippocampus and nicotine withdrawal deficits in learning. Identifying the factors underlying the withdrawal deficits should aid in improving therapeutics to treat nicotine addiction, but it is important to remember that nicotine addiction is a complex disorder with many factors influencing the disease progression. One factor that clearly contributes to the susceptibility and symptoms of nicotine withdrawal is genetics. Genetic background mediated the effects of both acute nicotine and withdrawal from chronic nicotine on learning in mice. Similarly, in humans, genetic polymorphisms are linked to multiple smoking phenotypes (see Portugal and Gould, 2008 for review). In addition to genetics, age also contributes to susceptibility to nicotine addiction. Adolescents appear to be more sensitive to the acute effects of nicotine and less sensitive to the immediate cognitive-related withdrawal symptoms. However, adolescents may be particularly sensitive to long-term detrimental effects of nicotine exposure compared to adults, as adolescent nicotine exposure was associated with the development of cognitive deficits in adulthood. This vulnerability attests to the importance of campaigns designed to reduce teen smoking and treat nicotine addiction in adolescents.
12.0 Future Directions
While advances have been made in understanding the neural effects of nicotine and the underlying neural substrates of nicotine addiction, many issues remain unresolved. One unresolved issue is where nicotine is acting in the hippocampus (i.e., DG, CA3, CA1) and acting at a cellular level in the hippocampus to alter learning. In the hippocampus, nAChR have been localized to pyramidal cells and also inhibitory interneurons (Alkondon and Albuquerque, 2001; 2004). Clearly, nicotinic modulation of pyramidal cell activity versus GABAergic interneurons will have a different effect on hippocampal signaling, and there is no guarantee that it is an either or situation; it is possible nicotine modulates a balance between pyramidal cells activity and inhibitory interneuron activity and that this contributes to the effects of nicotine on learning. In addition to the specific cell type involved in the effects of nicotine on learning, another unresolved issue is whether the effects of nicotine on learning occur directly through cholinergic signaling or involve modulation of the release of other neurotransmitters. Furthermore, whereas evidence suggests that nAChR upregulation may contribute to nicotine withdrawal deficits in cognition, it is unresolved whether these receptors are hyper or hypofunctional during withdrawal. Resolving these issues should improve pharmacotherapeutic development.
Finally, as reviewed, it is clear that genetics and developmental stage contribute to different effects of nicotine and regulate sensitivity to developing nicotine addiction. By understanding the genetic risk factors, treatments for nicotine addiction could be refined and tailored to genotype in order to increase effectiveness of therapies (Lerman, 2006). Adolescence is a time of high vulnerability to developing addiction and understanding the mechanism for this vulnerability may aid in reducing teenage smoking. Perhaps even more important, understanding how adolescent nicotine exposure contributes to adult depression (Iniguez et al., 2009) and cognitive impairments (Counotte et al., 2009) may lead to the development of interventions that reduce these effects and thus increase adult mental health.
Acknowledgments
Portions of the work reviewed here were supported by grants from the NIH: DA017949 (TJG), DA024787 (TJG), and CA143187 (Caryn Lerman). PTL was supported by NIH training grant: DA007237. The authors would also like to acknowledge Sheree Logue and Tatiana Kazdoba for critical reading of this manuscript.
Footnotes
Publisher's Disclaimer: This is a PDF file of an unedited manuscript that has been accepted for publication. As a service to our customers we are providing this early version of the manuscript. The manuscript will undergo copyediting, typesetting, and review of the resulting proof before it is published in its final citable form. Please note that during the production process errors may be discovered which could affect the content, and all legal disclaimers that apply to the journal pertain.
References
- Abdulla FA, Bradbury E, Calaminici MR, Lippiello PM, Wonnacott S, Gray JA, Sinden JD. Relationship between up-regulation of nicotine binding sites in rat brain and delayed cognitive enhancement observed after chronic or acute nicotinic receptor stimulation. Psychopharmacology (Berl) 1996;124:323–331. [Abstract] [Google Scholar]
- Abel T, Nguyen PV. Regulation of hippocampus-dependent memory by cyclic AMP-dependent protein kinase. Prog Brain Res. 2008;169:97–115. [Europe PMC free article] [Abstract] [Google Scholar]
- Abel T, Nguyen PV, Barad M, Deuel TA, Kandel ER, Bourtchouladze R. Genetic demonstration of a role for PKA in the late phase of LTP and in hippocampus-based long-term memory. Cell. 1997;88:615–626. [Abstract] [Google Scholar]
- Abood LG, Reynolds DT, Booth H, Bidlack JM. Sites and mechanisms for nicotine’s action in the brain. Neurosci Biobehav Rev. 1981;5:479–486. [Abstract] [Google Scholar]
- Abreu-Villaca Y, Seidler FJ, Slotkin TA. Impact of adolescent nicotine exposure on adenylyl cyclase-mediated cell signaling: enzyme induction, neurotransmitter-specific effects, regional selectivities, and the role of withdrawal. Brain Res. 2003;988:164–172. [Abstract] [Google Scholar]
- Abreu-Villaca Y, Seidler FJ, Tate CA, Slotkin TA. Nicotine is a neurotoxin in the adolescent brain: critical periods, patterns of exposure, regional selectivity, and dose thresholds for macromolecular alterations. Brain Res. 2003;979:114–128. [Abstract] [Google Scholar]
- Adler LE, Olincy A, Waldo M, Harris JG, Griffith J, Stevens K, Flach K, Nagamoto H, Bickford P, Leonard S, Freedman R. Schizophrenia, sensory gating, and nicotinic receptors. Schizophr Bull. 1998;24:189–202. [Abstract] [Google Scholar]
- Agster KL, Burwell RD. Cortical efferents of the perirhinal, postrhinal, and entorhinal cortices of the rat. Hippocampus. 2009;19:1159–1186. [Europe PMC free article] [Abstract] [Google Scholar]
- Akers RF, Lovinger DM, Colley PA, Linden DJ, Routtenberg A. Translocation of protein kinase C activity may mediate hippocampal long-term potentiation. Science. 1986;231:587–589. [Abstract] [Google Scholar]
- Alkondon M, Albuquerque EX. Nicotinic acetylcholine receptor alpha7 and alpha4beta2 subtypes differentially control GABAergic input to CA1 neurons in rat hippocampus. J Neurophysiol. 2001;86:3043–3055. [Abstract] [Google Scholar]
- Alkondon M, Albuquerque EX. The nicotinic acetylcholine receptor subtypes and their function in the hippocampus and cerebral cortex. Prog Brain Res. 2004;145:109–120. [Abstract] [Google Scholar]
- Alkondon M, Braga MF, Pereira EF, Maelicke A, Albuquerque EX. alpha7 nicotinic acetylcholine receptors and modulation of gabaergic synaptic transmission in the hippocampus. Eur J Pharmacol. 2000;393:59–67. [Abstract] [Google Scholar]
- Alkondon M, Pereira EF, Albuquerque EX. Mapping the location of functional nicotinic and gamma-aminobutyric acidA receptors on hippocampal neurons. J Pharmacol Exp Ther. 1996;279:1491–1506. [Abstract] [Google Scholar]
- Alkondon M, Pereira EF, Almeida LE, Randall WR, Albuquerque EX. Nicotine at concentrations found in cigarette smokers activates and desensitizes nicotinic acetylcholine receptors in CA1 interneurons of rat hippocampus. Neuropharmacology. 2000;39:2726–2739. [Abstract] [Google Scholar]
- Amar M, Thomas P, Johnson C, Lunt GG, Wonnacott S. Agonist pharmacology of the neuronal alpha 7 nicotinic receptor expressed in Xenopus oocytes. FEBS Lett. 1993;327:284–288. [Abstract] [Google Scholar]
- Amaral DG, Kurz J. An analysis of the origins of the cholinergic and noncholinergic septal projections to the hippocampal formation of the rat. J Comp Neurol. 1985;240:37–59. [Abstract] [Google Scholar]
- Amaral DG, Scharfman HE, Lavenex P. The dentate gyrus: fundamental neuroanatomical organization (dentate gyrus for dummies) Prog Brain Res. 2007;163:3–22. [Europe PMC free article] [Abstract] [Google Scholar]
- Anagnostaras SG, Maren S, Fanselow MS. Temporally graded retrograde amnesia of contextual fear after hippocampal damage in rats: within-subjects examination. J Neurosci. 1999;19:1106–1114. [Europe PMC free article] [Abstract] [Google Scholar]
- Andersen P, Bland BH, Dudar JD. Organization of the hippocampal output. Exp Brain Res. 1973;17:152–168. [Abstract] [Google Scholar]
- Andre JM, Gulick D, Portugal GS, Gould TJ. Nicotine withdrawal disrupts both foreground and background contextual fear conditioning but not pre-pulse inhibition of the acoustic startle response in C57BL/6 mice. Behav Brain Res. 2008;190:174–181. [Europe PMC free article] [Abstract] [Google Scholar]
- Andre JM, Leach PT, Gould TJ. Nicotine ameliorates NMDA receptor antagonist-induced deficits in contextual fear conditioning through high-affinity nicotinic acetylcholine receptors in the hippocampus. Neuropharmacology. 2011;60:617–625. [Europe PMC free article] [Abstract] [Google Scholar]
- Andrews JS, Jansen JH, Linders S, Princen A. Effects of disrupting the cholinergic system on short-term spatial memory in rats. Psychopharmacology (Berl) 1994;115:485–494. [Abstract] [Google Scholar]
- APA. Diagnostic and Statistical Manual of Mental Disorders. 4. Washington, DC: 2000. Text Revision (DSM-IV-TR) [Google Scholar]
- Araujo DM, Lapchak PA, Collier B, Quirion R. Characterization of N-[3H]methylcarbamylcholine binding sites and effect of N-methylcarbamylcholine on acetylcholine release in rat brain. J Neurochem. 1988;51:292–299. [Abstract] [Google Scholar]
- Arendt T, Allen Y, Marchbanks RM, Schugens MM, Sinden J, Lantos PL, Gray JA. Cholinergic system and memory in the rat: effects of chronic ethanol, embryonic basal forebrain brain transplants and excitotoxic lesions of cholinergic basal forebrain projection system. Neuroscience. 1989;33:435–462. [Abstract] [Google Scholar]
- Arqueros L, Naquira D, Zunino E. Nicotine-induced release of catecholamines from rat hippocampus and striatum. Biochem Pharmacol. 1978;27:2667–2674. [Abstract] [Google Scholar]
- Ascher P, Nowak L. The role of divalent cations in the N-methyl-D-aspartate responses of mouse central neurones in culture. J Physiol. 1988;399:247–266. [Abstract] [Google Scholar]
- Atkins CM, Selcher JC, Petraitis JJ, Trzaskos JM, Sweatt JD. The MAPK cascade is required for mammalian associative learning. Nat Neurosci. 1998;1:602–609. [Abstract] [Google Scholar]
- Attaway CM, Compton DM, Turner MD. The effects of nicotine on learning and memory: a neuropsychological assessment in young and senescent Fischer 344 rats. Physiol Behav. 1999;67:421–431. [Abstract] [Google Scholar]
- Azzopardi E, Typlt M, Jenkins B, Schmid S. Sensorimotor gating and spatial learning in alpha7-nicotinic receptor knockout mice. Genes Brain Behav. 2013;12:414–423. [Abstract] [Google Scholar]
- Babar E, Melik E, Ozgunen T. Excitotoxic median raphe lesions aggravate working memory storage performance deficits caused by scopolamine infusion into the dentate gyrus of the hippocampus in the inhibitory avoidance task in rats. Braz J Med Biol Res. 2002;35:479–484. [Abstract] [Google Scholar]
- Babar E, Melik E, Ozgunen T, Polat S. Effects of excitotoxic median raphe lesion on working memory deficits produced by the dorsal hippocampal muscarinic receptor blockade in the inhibitory avoidance in rats. Brain Res Bull. 2002;57:683–688. [Abstract] [Google Scholar]
- Bangasser DA, Waxler DE, Santollo J, Shors TJ. Trace conditioning and the hippocampus: the importance of contiguity. J Neurosci. 2006;26:8702–8706. [Europe PMC free article] [Abstract] [Google Scholar]
- Bannerman DM, Grubb M, Deacon RM, Yee BK, Feldon J, Rawlins JN. Ventral hippocampal lesions affect anxiety but not spatial learning. Behav Brain Res. 2003;139:197–213. [Abstract] [Google Scholar]
- Barbosa FF, Pontes IM, Ribeiro S, Ribeiro AM, Silva RH. Differential roles of the dorsal hippocampal regions in the acquisition of spatial and temporal aspects of episodic-like memory. Behav Brain Res. 2012;232:269–277. [Abstract] [Google Scholar]
- Bardgett ME, Henry JD. Locomotor activity and accumbens Fos expression driven by ventral hippocampal stimulation require D1 and D2 receptors. Neuroscience. 1999;94:59–70. [Abstract] [Google Scholar]
- Barik J, Wonnacott S. Indirect modulation by alpha7 nicotinic acetylcholine receptors of noradrenaline release in rat hippocampal slices: interaction with glutamate and GABA systems and effect of nicotine withdrawal. Mol Pharmacol. 2006;69:618–628. [Abstract] [Google Scholar]
- Barker GR, Warburton EC. When is the hippocampus involved in recognition memory? J Neurosci. 2011;31:10721–10731. [Europe PMC free article] [Abstract] [Google Scholar]
- Baron SP, Wright D, Wenger GR. Effects of drugs of abuse and scopolamine on memory in rats: delayed spatial alternation and matching to position. Psychopharmacology (Berl) 1998;137:7–14. [Abstract] [Google Scholar]
- Barrantes GE, Rogers AT, Lindstrom J, Wonnacott S. alpha-Bungarotoxin binding sites in rat hippocampal and cortical cultures: initial characterisation, colocalisation with alpha 7 subunits and up-regulation by chronic nicotine treatment. Brain Res. 1995;672:228–236. [Abstract] [Google Scholar]
- Bartesaghi R, Gessi T, Migliore M. Input-output relations in the entorhinal-hippocampal-entorhinal loop: entorhinal cortex and dentate gyrus. Hippocampus. 1995;5:440–451. [Abstract] [Google Scholar]
- Bast T, Zhang WN, Feldon J. The ventral hippocampus and fear conditioning in rats. Different anterograde amnesias of fear after tetrodotoxin inactivation and infusion of the GABA(A) agonist muscimol. Exp Brain Res. 2001;139:39–52. [Abstract] [Google Scholar]
- Bast T, Zhang WN, Heidbreder C, Feldon J. Hyperactivity and disruption of prepulse inhibition induced by N-methyl-D-aspartate stimulation of the ventral hippocampus and the effects of pretreatment with haloperidol and clozapine. Neuroscience. 2001;103:325–335. [Abstract] [Google Scholar]
- Belin D, Jonkman S, Dickinson A, Robbins TW, Everitt BJ. Parallel and interactive learning processes within the basal ganglia: relevance for the understanding of addiction. Behav Brain Res. 2009;199:89–102. [Abstract] [Google Scholar]
- Bell SL, Taylor RC, Singleton EG, Henningfield JE, Heishman SJ. Smoking after nicotine deprivation enhances cognitive performance and decreases tobacco craving in drug abusers. Nicotine Tob Res. 1999;1:45–52. [Abstract] [Google Scholar]
- Benes FM, Kwok EW, Vincent SL, Todtenkopf MS. A reduction of nonpyramidal cells in sector CA2 of schizophrenics and manic depressives. Biol Psychiatry. 1998;44:88–97. [Abstract] [Google Scholar]
- Bennett MR, Gibson WG, Robinson J. Dynamics of the CA3 pyramidal neuron autoassociative memory network in the hippocampus. Philos Trans R Soc Lond B Biol Sci. 1994;343:167–187. [Abstract] [Google Scholar]
- Berke JD, Hyman SE. Addiction, dopamine, and the molecular mechanisms of memory. Neuron. 2000;25:515–532. [Abstract] [Google Scholar]
- Bernabeu R, Bevilaqua L, Ardenghi P, Bromberg E, Schmitz P, Bianchin M, Izquierdo I, Medina JH. Involvement of hippocampal cAMP/cAMP-dependent protein kinase signaling pathways in a late memory consolidation phase of aversively motivated learning in rats. Proc Natl Acad Sci U S A. 1997;94:7041–7046. [Europe PMC free article] [Abstract] [Google Scholar]
- Bernal MC, Vicens P, Carrasco MC, Redolat R. Effects of nicotine on spatial learning in C57BL mice. Behav Pharmacol. 1999;10:333–336. [Abstract] [Google Scholar]
- Beselia G, Maglakelidze G, Chkhikvishvili N, Burjanadze M, Dashniani M. Georgian Med News. 2010. Object exploration and reactions to spatial and nonspatial changes in dentate gyrus lesioned rats; pp. 61–65. [Abstract] [Google Scholar]
- Bevins RA, Besheer J. Object recognition in rats and mice: a one-trial non-matching-to-sample learning task to study ‘recognition memory’ Nat Protoc. 2006;1:1306–1311. [Abstract] [Google Scholar]
- Bigl V, Woolf NJ, Butcher LL. Cholinergic projections from the basal forebrain to frontal, parietal, temporal, occipital, and cingulate cortices: a combined fluorescent tracer and acetylcholinesterase analysis. Brain Res Bull. 1982;8:727–749. [Abstract] [Google Scholar]
- Bjorkblom B, Ostman N, Hongisto V, Komarovski V, Filen JJ, Nyman TA, Kallunki T, Courtney MJ, Coffey ET. Constitutively active cytoplasmic c-Jun N-terminal kinase 1 is a dominant regulator of dendritic architecture: role of microtubule-associated protein 2 as an effector. J Neurosci. 2005;25:6350–6361. [Europe PMC free article] [Abstract] [Google Scholar]
- Blackstad TW. Commissural connections of the hippocampal region in the rat, with special reference to their mode of termination. J Comp Neurol. 1956;105:417–537. [Abstract] [Google Scholar]
- Blakemore SJ, Robbins TW. Decision-making in the adolescent brain. Nat Neurosci. 2012;15:1184–1191. [Abstract] [Google Scholar]
- Bliss TV, Collingridge GL. A synaptic model of memory: long-term potentiation in the hippocampus. Nature. 1993;361:31–39. [Abstract] [Google Scholar]
- Bliss TV, Gardner-Medwin AR. Long-lasting potentiation of synaptic transmission in the dentate area of the unanaestetized rabbit following stimulation of the perforant path. J Physiol. 1973;232:357–374. [Abstract] [Google Scholar]
- Bliss TV, Lomo T. Long-lasting potentiation of synaptic transmission in the dentate area of the anaesthetized rabbit following stimulation of the perforant path. J Physiol. 1973;232:331–356. [Abstract] [Google Scholar]
- Blumenfeld RS, Ranganath C. Prefrontal cortex and long-term memory encoding: an integrative review of findings from neuropsychology and neuroimaging. Neuroscientist. 2007;13:280–291. [Abstract] [Google Scholar]
- Bogoyevitch MA, Kobe B. Uses for JNK: the many and varied substrates of the c-Jun N-terminal kinases. Microbiol Mol Biol Rev. 2006;70:1061–1095. [Europe PMC free article] [Abstract] [Google Scholar]
- Bontempi B, Laurent-Demir C, Destrade C, Jaffard R. Time-dependent reorganization of brain circuitry underlying long-term memory storage. Nature. 1999;400:671–675. [Abstract] [Google Scholar]
- Borhegyi Z, Leranth C. Substance P innervation of the rat hippocampal formation. J Comp Neurol. 1997;384:41–58. [Abstract] [Google Scholar]
- Borrelli E, Montmayeur JP, Foulkes NS, Sassone-Corsi P. Signal transduction and gene control: the cAMP pathway. Crit Rev Oncog. 1992;3:321–338. [Abstract] [Google Scholar]
- Bourtchuladze R, Frenguelli B, Blendy J, Cioffi D, Schutz G, Silva AJ. Deficient long-term memory in mice with a targeted mutation of the cAMP-responsive element-binding protein. Cell. 1994;79:59–68. [Abstract] [Google Scholar]
- Bremner JD, Narayan M, Anderson ER, Staib LH, Miller HL, Charney DS. Hippocampal volume reduction in major depression. Am J Psychiatry. 2000;157:115–118. [Abstract] [Google Scholar]
- Breslau N, Peterson EL. Smoking cessation in young adults: age at initiation of cigarette smoking and other suspected influences. Am J Public Health. 1996;86:214–220. [Abstract] [Google Scholar]
- Broadbent NJ, Gaskin S, Squire LR, Clark RE. Object recognition memory and the rodent hippocampus. Learn Mem. 2009;17:5–11. [Europe PMC free article] [Abstract] [Google Scholar]
- Brown DA. Muscarinic acetylcholine receptors (mAChRs) in the nervous system: some functions and mechanisms. J Mol Neurosci. 2010;41:340–346. [Abstract] [Google Scholar]
- Brown DA, Abogadie FC, Allen TG, Buckley NJ, Caulfield MP, Delmas P, Haley JE, Lamas JA, Selyanko AA. Muscarinic mechanisms in nerve cells. Life Sci. 1997;60:1137–1144. [Abstract] [Google Scholar]
- Burwell RD, Amaral DG. Perirhinal and postrhinal cortices of the rat: interconnectivity and connections with the entorhinal cortex. J Comp Neurol. 1998;391:293–321. [Abstract] [Google Scholar]
- Bussey TJ, Duck J, Muir JL, Aggleton JP. Distinct patterns of behavioural impairments resulting from fornix transection or neurotoxic lesions of the perirhinal and postrhinal cortices in the rat. Behav Brain Res. 2000;111:187–202. [Abstract] [Google Scholar]
- Caldarone BJ, Duman CH, Picciotto MR. Fear conditioning and latent inhibition in mice lacking the high affinity subclass of nicotinic acetylcholine receptors in the brain. Neuropharmacology. 2000;39:2779–2784. [Abstract] [Google Scholar]
- Canteras NS, Swanson LW. Projections of the ventral subiculum to the amygdala, septum, and hypothalamus: a PHAL anterograde tract-tracing study in the rat. J Comp Neurol. 1992;324:180–194. [Abstract] [Google Scholar]
- Casey BJ, Jones RM, Hare TA. The adolescent brain. Ann N Y Acad Sci. 2008;1124:111–126. [Europe PMC free article] [Abstract] [Google Scholar]
- Casey BJ, Tottenham N, Liston C, Durston S. Imaging the developing brain: what have we learned about cognitive development? Trends Cogn Sci. 2005;9:104–110. [Abstract] [Google Scholar]
- Caulfield MP, Robbins J, Higashida H, Brown DA. Postsynaptic actions of acetylcholine: the coupling of muscarinic receptor subtypes to neuronal ion channels. Prog Brain Res. 1993;98:293–301. [Abstract] [Google Scholar]
- Cenquizca LA, Swanson LW. Spatial organization of direct hippocampal field CA1 axonal projections to the rest of the cerebral cortex. Brain Res Rev. 2007;56:1–26. [Europe PMC free article] [Abstract] [Google Scholar]
- Chang L, Jones Y, Ellisman MH, Goldstein LS, Karin M. JNK1 is required for maintenance of neuronal microtubules and controls phosphorylation of microtubule-associated proteins. Dev Cell. 2003;4:521–533. [Abstract] [Google Scholar]
- Chavez-Noriega LE, Crona JH, Washburn MS, Urrutia A, Elliott KJ, Johnson EC. Pharmacological characterization of recombinant human neuronal nicotinic acetylcholine receptors h alpha 2 beta 2, h alpha 2 beta 4, h alpha 3 beta 2, h alpha 3 beta 4, h alpha 4 beta 2, h alpha 4 beta 4 and h alpha 7 expressed in Xenopus oocytes. J Pharmacol Exp Ther. 1997;280:346–356. [Abstract] [Google Scholar]
- Chen D, Patrick JW. The alpha-bungarotoxin-binding nicotinic acetylcholine receptor from rat brain contains only the alpha7 subunit. J Biol Chem. 1997;272:24024–24029. [Abstract] [Google Scholar]
- Chevaleyre V, Siegelbaum SA. Strong CA2 pyramidal neuron synapses define a powerful disynaptic cortico-hippocampal loop. Neuron. 2010;66:560–572. [Europe PMC free article] [Abstract] [Google Scholar]
- Clarke PB, Fibiger HC. Reinforced alternation performance is impaired by muscarinic but not by nicotinic receptor blockade in rats. Behav Brain Res. 1990;36:203–207. [Abstract] [Google Scholar]
- Clarke PB, Reuben M. Release of [3H]-noradrenaline from rat hippocampal synaptosomes by nicotine: mediation by different nicotinic receptor subtypes from striatal [3H]-dopamine release. Br J Pharmacol. 1996;117:595–606. [Europe PMC free article] [Abstract] [Google Scholar]
- Clarke PB, Schwartz RD, Paul SM, Pert CB, Pert A. Nicotinic binding in rat brain: autoradiographic comparison of [3H]acetylcholine, [3H]nicotine, and [125I]-alpha-bungarotoxin. J Neurosci. 1985;5:1307–1315. [Europe PMC free article] [Abstract] [Google Scholar]
- Cole AE, Nicoll RA. The pharmacology of cholinergic excitatory responses in hippocampal pyramidal cells. Brain Res. 1984;305:283–290. [Abstract] [Google Scholar]
- Colgin LL, Kubota D, Jia Y, Rex CS, Lynch G. Long-term potentiation is impaired in rat hippocampal slices that produce spontaneous sharp waves. J Physiol. 2004;558:953–961. [Abstract] [Google Scholar]
- Collingridge GL, Kehl SJ, McLennan H. Excitatory amino acids in synaptic transmission in the Schaffer collateral-commissural pathway of the rat hippocampus. J Physiol. 1983;334:33–46. [Abstract] [Google Scholar]
- Compton DM. Encoding of a nonmonotonic serial pattern: role of the dorsal hippocampus and amygdala. Physiol Behav. 1993;53:657–665. [Abstract] [Google Scholar]
- Corringer PJ, Le Novere N, Changeux JP. Nicotinic receptors at the amino acid level. Annu Rev Pharmacol Toxicol. 2000;40:431–458. [Abstract] [Google Scholar]
- Costa LG, Murphy SD. [3H]Nicotine binding in rat brain: alteration after chronic acetylcholinesterase inhibition. J Pharmacol Exp Ther. 1983;226:392–397. [Abstract] [Google Scholar]
- Costa VC, Bueno JL, Xavier GF. Dentate gyrus-selective colchicine lesion and performance in temporal and spatial tasks. Behav Brain Res. 2005;160:286–303. [Abstract] [Google Scholar]
- Counotte DS, Spijker S, Van de Burgwal LH, Hogenboom F, Schoffelmeer AN, De Vries TJ, Smit AB, Pattij T. Long-lasting cognitive deficits resulting from adolescent nicotine exposure in rats. Neuropsychopharmacology. 2009;34:299–306. [Abstract] [Google Scholar]
- Cravens CJ, Vargas-Pinto N, Christian KM, Nakazawa K. CA3 NMDA receptors are crucial for rapid and automatic representation of context memory. Eur J Neurosci. 2006;24:1771–1780. [Abstract] [Google Scholar]
- Crutcher KA, Madison R, Davis JN. A study of the rat septohippocampal pathway using anterograde transport of horseradish peroxidase. Neuroscience. 1981;6:1961–1973. [Abstract] [Google Scholar]
- Cui Z, Gerfen CR, Young WS., 3rd Hypothalamic and other connections with the dorsal CA2 area of the mouse hippocampus. J Comp Neurol 2012 [Europe PMC free article] [Abstract] [Google Scholar]
- Czerniawski J, Ree F, Chia C, Otto T. Dorsal versus ventral hippocampal contributions to trace and contextual conditioning: differential effects of regionally selective NMDA receptor antagonism on acquisition and expression. Hippocampus. 2012;22:1528–1539. [Abstract] [Google Scholar]
- Czerniawski J, Yoon T, Otto T. Dissociating space and trace in dorsal and ventral hippocampus. Hippocampus. 2009;19:20–32. [Abstract] [Google Scholar]
- Dani JA, Heinemann S. Molecular and cellular aspects of nicotine abuse. Neuron. 1996;16:905–908. [Abstract] [Google Scholar]
- Daumas S, Ceccom J, Halley H, Frances B, Lassalle JM. Activation of metabotropic glutamate receptor type 2/3 supports the involvement of the hippocampal mossy fiber pathway on contextual fear memory consolidation. Learn Mem. 2009;16:504–507. [Abstract] [Google Scholar]
- Daumas S, Halley H, Frances B, Lassalle JM. Encoding, consolidation, and retrieval of contextual memory: differential involvement of dorsal CA3 and CA1 hippocampal subregions. Learn Mem. 2005;12:375–382. [Europe PMC free article] [Abstract] [Google Scholar]
- Daumas S, Halley H, Lassalle JM. Disruption of hippocampal CA3 network: effects on episodic-like memory processing in C57BL/6J mice. Eur J Neurosci. 2004;20:597–600. [Abstract] [Google Scholar]
- Davidson RJ, Pizzagalli D, Nitschke JB, Putnam K. Depression: perspectives from affective neuroscience. Annu Rev Psychol. 2002;53:545–574. [Abstract] [Google Scholar]
- Davis JA, Gould TJ. The effects of DHBE and MLA on nicotine-induced enhancement of contextual fear conditioning in C57BL/6 mice. Psychopharmacology (Berl) 2006;184:345–352. [Abstract] [Google Scholar]
- Davis JA, Gould TJ. Atomoxetine reverses nicotine withdrawal-associated deficits in contextual fear conditioning. Neuropsychopharmacology. 2007a;32:2011–2019. [Europe PMC free article] [Abstract] [Google Scholar]
- Davis JA, Gould TJ. beta2 subunit-containing nicotinic receptors mediate the enhancing effect of nicotine on trace cued fear conditioning in C57BL/6 mice. Psychopharmacology (Berl) 2007b;190:343–352. [Europe PMC free article] [Abstract] [Google Scholar]
- Davis JA, Gould TJ. Hippocampal nAChRs mediate nicotine withdrawal-related learning deficits. Eur Neuropsychopharmacol. 2009;19:551–561. [Europe PMC free article] [Abstract] [Google Scholar]
- Davis JA, James JR, Siegel SJ, Gould TJ. Withdrawal from chronic nicotine administration impairs contextual fear conditioning in C57BL/6 mice. J Neurosci. 2005;25:8708–8713. [Europe PMC free article] [Abstract] [Google Scholar]
- Davis JA, Kenney JW, Gould TJ. Hippocampal alpha4beta2 nicotinic acetylcholine receptor involvement in the enhancing effect of acute nicotine on contextual fear conditioning. J Neurosci. 2007;27:10870–10877. [Europe PMC free article] [Abstract] [Google Scholar]
- Davis JA, Porter J, Gould TJ. Nicotine enhances both foreground and background contextual fear conditioning. Neurosci Lett. 2006;394:202–205. [Europe PMC free article] [Abstract] [Google Scholar]
- DeBry SC, Tiffany ST. Tobacco-induced neurotoxicity of adolescent cognitive development (TINACD): a proposed model for the development of impulsivity in nicotine dependence. Nicotine Tob Res. 2008;10:11–25. [Abstract] [Google Scholar]
- Decker MW, Majchrzak MJ. Effects of systemic and intracerebroventricular administration of mecamylamine, a nicotinic cholinergic antagonist, on spatial memory in rats. Psychopharmacology (Berl) 1992;107:530–534. [Abstract] [Google Scholar]
- Delgado MR. Reward-related responses in the human striatum. Ann N Y Acad Sci. 2007;1104:70–88. [Abstract] [Google Scholar]
- Dennis M, Giraudat J, Kotzyba-Hibert F, Goeldner M, Hirth C, Chang JY, Lazure C, Chretien M, Changeux JP. Amino acids of the Torpedo marmorata acetylcholine receptor alpha subunit labeled by a photoaffinity ligand for the acetylcholine binding site. Biochemistry. 1988;27:2346–2357. [Abstract] [Google Scholar]
- Dere E, Huston JP, De Souza Silva MA. The pharmacology, neuroanatomy and neurogenetics of one-trial object recognition in rodents. Neurosci Biobehav Rev. 2007;31:673–704. [Abstract] [Google Scholar]
- Dere E, Pause BM, Pietrowsky R. Emotion and episodic memory in neuropsychiatric disorders. Behav Brain Res. 2010;215:162–171. [Abstract] [Google Scholar]
- Deshmukh SS, Knierim JJ. Representation of non-spatial and spatial information in the lateral entorhinal cortex. Front Behav Neurosci. 2011;5:69. [Europe PMC free article] [Abstract] [Google Scholar]
- Deutsch JA. The cholinergic synapse and the site of memory. Science. 1971;174:788–794. [Abstract] [Google Scholar]
- Di Chiara G. Nucleus accumbens shell and core dopamine: differential role in behavior and addiction. Behav Brain Res. 2002;137:75–114. [Abstract] [Google Scholar]
- Dilts SL. Berry CA: Effect of cholinergic drugs on passive avoidance in the mouse. J Pharmacol Exp Ther. 1967;158:279–285. [Abstract] [Google Scholar]
- Dominguez del Toro E, Juiz JM, Peng X, Lindstrom J, Criado M. Immunocytochemical localization of the alpha 7 subunit of the nicotinic acetylcholine receptor in the rat central nervous system. J Comp Neurol. 1994;349:325–342. [Abstract] [Google Scholar]
- Due DL, Huettel SA, Hall WG, Rubin DC. Activation in mesolimbic and visuospatial neural circuits elicited by smoking cues: evidence from functional magnetic resonance imaging. Am J Psychiatry. 2002;159:954–960. [Abstract] [Google Scholar]
- Duman RS, Heninger GR, Nestler EJ. A molecular and cellular theory of depression. Arch Gen Psychiatry. 1997;54:597–606. [Abstract] [Google Scholar]
- Duman RS, Voleti B. Signaling pathways underlying the pathophysiology and treatment of depression: novel mechanisms for rapid-acting agents. Trends Neurosci. 2012;35:47–56. [Europe PMC free article] [Abstract] [Google Scholar]
- Dzidzishvili NN, Ungiadze AA, Davituliani D. The effect of dorsal and ventral hippocampal lesions on short-term memory in cats. Zh Vyssh Nerv Deiat Im I P Pavlova. 1975;25:70–77. [Abstract] [Google Scholar]
- Empson RM, Heinemann U. The perforant path projection to hippocampal area CA1 in the rat hippocampal-entorhinal cortex combined slice. J Physiol. 1995;484(Pt 3):707–720. [Abstract] [Google Scholar]
- English JD, Sweatt JD. A requirement for the mitogen-activated protein kinase cascade in hippocampal long term potentiation. J Biol Chem. 1997;272:19103–19106. [Abstract] [Google Scholar]
- Ennaceur A. One-trial object recognition in rats and mice: methodological and theoretical issues. Behav Brain Res. 2010;215:244–254. [Abstract] [Google Scholar]
- Ennaceur A, Neave N, Aggleton JP. Spontaneous object recognition and object location memory in rats: the effects of lesions in the cingulate cortices, the medial prefrontal cortex, the cingulum bundle and the fornix. Exp Brain Res. 1997;113:509–519. [Abstract] [Google Scholar]
- Esclassan F, Coutureau E, Di Scala G, Marchand AR. A cholinergic-dependent role for the entorhinal cortex in trace fear conditioning. J Neurosci. 2009;29:8087–8093. [Europe PMC free article] [Abstract] [Google Scholar]
- Estus S, Zaks WJ, Freeman RS, Gruda M, Bravo R, Johnson EM., Jr Altered gene expression in neurons during programmed cell death: identification of c-jun as necessary for neuronal apoptosis. J Cell Biol. 1994;127:1717–1727. [Europe PMC free article] [Abstract] [Google Scholar]
- Fabian-Fine R, Skehel P, Errington ML, Davies HA, Sher E, Stewart MG, Fine A. Ultrastructural distribution of the alpha7 nicotinic acetylcholine receptor subunit in rat hippocampus. J Neurosci. 2001;21:7993–8003. [Europe PMC free article] [Abstract] [Google Scholar]
- Fanselow MS. Factors governing one-trial contextual conditioning. Animal Learning & Behavior. 1990;18:264–270. [Google Scholar]
- Fanselow MS, Dong HW. Are the dorsal and ventral hippocampus functionally distinct structures? Neuron. 2010;65:7–19. [Europe PMC free article] [Abstract] [Google Scholar]
- Fanselow MS, Kim JJ, Yipp J, De Oca B. Differential effects of the N-methyl-D-aspartate antagonist DL-2-amino-5-phosphonovalerate on acquisition of fear of auditory and contextual cues. Behav Neurosci. 1994;108:235–240. [Abstract] [Google Scholar]
- Feiro O, Gould TJ. The interactive effects of nicotinic and muscarinic cholinergic receptor inhibition on fear conditioning in young and aged C57BL/6 mice. Pharmacol Biochem Behav. 2005;80:251–262. [Abstract] [Google Scholar]
- Ferbinteanu J, Ray C, McDonald RJ. Both dorsal and ventral hippocampus contribute to spatial learning in Long-Evans rats. Neurosci Lett. 2003;345:131–135. [Abstract] [Google Scholar]
- Flavell CR, Lee JL. Post-training unilateral amygdala lesions selectively impair contextual fear memories. Learn Mem. 2012;19:256–263. [Abstract] [Google Scholar]
- Florian C, Roullet P. Hippocampal CA3-region is crucial for acquisition and memory consolidation in Morris water maze task in mice. Behav Brain Res. 2004;154:365–374. [Abstract] [Google Scholar]
- Forwood SE, Winters BD, Bussey TJ. Hippocampal lesions that abolish spatial maze performance spare object recognition memory at delays of up to 48 hours. Hippocampus. 2005;15:347–355. [Abstract] [Google Scholar]
- Franklin TR, Wang Z, Wang J, Sciortino N, Harper D, Li Y, Ehrman R, Kampman K, O’Brien CP, Detre JA, Childress AR. Limbic activation to cigarette smoking cues independent of nicotine withdrawal: a perfusion fMRI study. Neuropsychopharmacology. 2007;32:2301–2309. [Abstract] [Google Scholar]
- Freedman R, Hall M, Adler LE, Leonard S. Evidence in postmortem brain tissue for decreased numbers of hippocampal nicotinic receptors in schizophrenia. Biol Psychiatry. 1995;38:22–33. [Abstract] [Google Scholar]
- Frey U, Frey S, Schollmeier F, Krug M. Influence of actinomycin D, a RNA synthesis inhibitor, on long-term potentiation in rat hippocampal neurons in vivo and in vitro. J Physiol. 1996;490(Pt 3):703–711. [Abstract] [Google Scholar]
- Frey U, Krug M, Reymann KG, Matthies H. Anisomycin, an inhibitor of protein synthesis, blocks late phases of LTP phenomena in the hippocampal CA1 region in vitro. Brain Res. 1988;452:57–65. [Abstract] [Google Scholar]
- Fricke R, Cowan WM. An autoradiographic study of the commissural and ipsilateral hippocampo-dentate projections in the adult rat. J Comp Neurol. 1978;181:253–269. [Abstract] [Google Scholar]
- Fryer JD, Lukas RJ. Noncompetitive functional inhibition at diverse, human nicotinic acetylcholine receptor subtypes by bupropion, phencyclidine, and ibogaine. J Pharmacol Exp Ther. 1999;288:88–92. [Abstract] [Google Scholar]
- Fuchs RA, Evans KA, Ledford CC, Parker MP, Case JM, Mehta RH, See RE. The role of the dorsomedial prefrontal cortex, basolateral amygdala, and dorsal hippocampus in contextual reinstatement of cocaine seeking in rats. Neuropsychopharmacology. 2005;30:296–309. [Abstract] [Google Scholar]
- Fujii S, Ji Z, Morita N, Sumikawa K. Acute and chronic nicotine exposure differentially facilitate the induction of LTP. Brain Res. 1999;846:137–143. [Abstract] [Google Scholar]
- Fujii S, Ji Z, Sumikawa K. Inactivation of alpha7 ACh receptors and activation of non-alpha7 ACh receptors both contribute to long term potentiation induction in the hippocampal CA1 region. Neurosci Lett. 2000;286:134–138. [Abstract] [Google Scholar]
- Fyhn M, Molden S, Witter MP, Moser EI, Moser MB. Spatial representation in the entorhinal cortex. Science. 2004;305:1258–1264. [Abstract] [Google Scholar]
- Gahring LC, Persiyanov K, Dunn D, Weiss R, Meyer EL, Rogers SW. Mouse strain-specific nicotinic acetylcholine receptor expression by inhibitory interneurons and astrocytes in the dorsal hippocampus. J Comp Neurol. 2004;468:334–346. [Abstract] [Google Scholar]
- Gahring LC, Rogers SW. Nicotinic acetylcholine receptor expression in the hippocampus of 27 mouse strains reveals novel inhibitory circuitry. Hippocampus. 2008;18:737–749. [Europe PMC free article] [Abstract] [Google Scholar]
- Ge S, Dani JA. Nicotinic acetylcholine receptors at glutamate synapses facilitate long-term depression or potentiation. J Neurosci. 2005;25:6084–6091. [Europe PMC free article] [Abstract] [Google Scholar]
- Gilbert PE, Kesner RP. The role of the dorsal CA3 hippocampal subregion in spatial working memory and pattern separation. Behav Brain Res. 2006;169:142–149. [Abstract] [Google Scholar]
- Gilbert PE, Kesner RP, Lee I. Dissociating hippocampal subregions: double dissociation between dentate gyrus and CA1. Hippocampus. 2001;11:626–636. [Abstract] [Google Scholar]
- Gilmartin MR, Kwapis JL, Helmstetter FJ. Trace and contextual fear conditioning are impaired following unilateral microinjection of muscimol in the ventral hippocampus or amygdala, but not the medial prefrontal cortex. Neurobiol Learn Mem. 2012;97:452–464. [Europe PMC free article] [Abstract] [Google Scholar]
- Gitelman DR, Prohovnik I. Muscarinic and nicotinic contributions to cognitive function and cortical blood flow. Neurobiol Aging. 1992;13:313–318. [Abstract] [Google Scholar]
- Gonzales D, Rennard SI, Nides M, Oncken C, Azoulay S, Billing CB, Watsky EJ, Gong J, Williams KE, Reeves KR. Varenicline, an alpha4beta2 nicotinic acetylcholine receptor partial agonist, vs sustained-release bupropion and placebo for smoking cessation: a randomized controlled trial. Jama. 2006;296:47–55. [Abstract] [Google Scholar]
- Gotti C, Clementi F, Fornari A, Gaimarri A, Guiducci S, Manfredi I, Moretti M, Pedrazzi P, Pucci L, Zoli M. Structural and functional diversity of native brain neuronal nicotinic receptors. Biochem Pharmacol. 2009;78:703–711. [Abstract] [Google Scholar]
- Gould TJ. Addiction and cognition. Addict Sci Clin Pract. 2010;5:4–14. [Europe PMC free article] [Abstract] [Google Scholar]
- Gould TJ, Feiro O, Moore D. Nicotine enhances trace cued fear conditioning but not delay cued fear conditioning in C57BL/6 mice. Behav Brain Res. 2004;155:167–173. [Abstract] [Google Scholar]
- Gould TJ, Higgins JS. Nicotine enhances contextual fear conditioning in C57BL/6J mice at 1 and 7 days post-training. Neurobiol Learn Mem. 2003;80:147–157. [Abstract] [Google Scholar]
- Gould TJ, Lewis MC. Coantagonism of glutamate receptors and nicotinic acetylcholinergic receptors disrupts fear conditioning and latent inhibition of fear conditioning. Learn Mem. 2005;12:389–398. [Europe PMC free article] [Abstract] [Google Scholar]
- Gould TJ, Lommock JA. Nicotine enhances contextual fear conditioning and ameliorates ethanol-induced deficits in contextual fear conditioning. Behav Neurosci. 2003;117:1276–1282. [Abstract] [Google Scholar]
- Gould TJ, McCarthy MM, Keith RA. MK-801 disrupts acquisition of contextual fear conditioning but enhances memory consolidation of cued fear conditioning. Behav Pharmacol. 2002;13:287–294. [Abstract] [Google Scholar]
- Gould TJ, Portugal GS, Andre JM, Tadman MP, Marks MJ, Kenney JW, Yildirim E, Adoff M. The duration of nicotine withdrawal-associated deficits in contextual fear conditioning parallels changes in hippocampal high affinity nicotinic acetylcholine receptor upregulation. Neuropharmacology. 2012;62:2118–2125. [Europe PMC free article] [Abstract] [Google Scholar]
- Gould TJ, Wehner JM. Nicotine enhancement of contextual fear conditioning. Behav Brain Res. 1999;102:31–39. [Abstract] [Google Scholar]
- Graef S, Schonknecht P, Sabri O, Hegerl U. Cholinergic receptor subtypes and their role in cognition, emotion, and vigilance control: an overview of preclinical and clinical findings. Psychopharmacology (Berl) 2011;215:205–229. [Abstract] [Google Scholar]
- Gravius A, Barberi C, Schafer D, Schmidt WJ, Danysz W. The role of group I metabotropic glutamate receptors in acquisition and expression of contextual and auditory fear conditioning in rats - a comparison. Neuropharmacology. 2006;51:1146–1155. [Abstract] [Google Scholar]
- Gray R, Rajan AS, Radcliffe KA, Yakehiro M, Dani JA. Hippocampal synaptic transmission enhanced by low concentrations of nicotine. Nature. 1996;383:713–716. [Abstract] [Google Scholar]
- Groenewegen HJ, Vermeulen-Van der Zee E, te Kortschot A, Witter MP. Organization of the projections from the subiculum to the ventral striatum in the rat. A study using anterograde transport of Phaseolus vulgaris leucoagglutinin. Neuroscience. 1987;23:103–120. [Abstract] [Google Scholar]
- Grundey J, Thirugnanasambandam N, Kaminsky K, Drees A, Skwirba AC, Lang N, Paulus W, Nitsche MA. Neuroplasticity in cigarette smokers is altered under withdrawal and partially restituted by nicotine exposition. J Neurosci. 2012;32:4156–4162. [Europe PMC free article] [Abstract] [Google Scholar]
- Gulick D, Gould TJ. Interactive effects of ethanol and nicotine on learning in C57BL/6J mice depend on both dose and duration of treatment. Psychopharmacology (Berl) 2008;196:483–495. [Europe PMC free article] [Abstract] [Google Scholar]
- Gupta S, Barrett T, Whitmarsh AJ, Cavanagh J, Sluss HK, Derijard B, Davis RJ. Selective interaction of JNK protein kinase isoforms with transcription factors. Embo J. 1996;15:2760–2770. [Europe PMC free article] [Abstract] [Google Scholar]
- Handelmann GE, Olton DS. Spatial memory following damage to hippocampal CA3 pyramidal cells with kainic acid: impairment and recovery with preoperative training. Brain Res. 1981;217:41–58. [Abstract] [Google Scholar]
- Harris EW, Cotman CW. Long-term potentiation of guinea pig mossy fiber responses is not blocked by N-methyl D-aspartate antagonists. Neurosci Lett. 1986;70:132–137. [Abstract] [Google Scholar]
- Harvey AL. The pharmacology of galanthamine and its analogues. Pharmacol Ther. 1995;68:113–128. [Abstract] [Google Scholar]
- Harvey SC, Luetje CW. Determinants of competitive antagonist sensitivity on neuronal nicotinic receptor beta subunits. J Neurosci. 1996;16:3798–3806. [Europe PMC free article] [Abstract] [Google Scholar]
- Harvey SC, Maddox FN, Luetje CW. Multiple determinants of dihydro-beta-erythroidine sensitivity on rat neuronal nicotinic receptor alpha subunits. J Neurochem. 1996;67:1953–1959. [Abstract] [Google Scholar]
- Hassall CJ, Stanford SC, Burnstock G, Buckley NJ. Co-expression of four muscarinic receptor genes by the intrinsic neurons of the rat and guinea-pig heart. Neuroscience. 1993;56:1041–1048. [Abstract] [Google Scholar]
- Hasselmo ME. Neuromodulation and cortical function: modeling the physiological basis of behavior. Behav Brain Res. 1995;67:1–27. [Abstract] [Google Scholar]
- Hasselmo ME. What is the function of hippocampal theta rhythm?--Linking behavioral data to phasic properties of field potential and unit recording data. Hippocampus. 2005;15:936–949. [Abstract] [Google Scholar]
- Hasselmo ME. The role of acetylcholine in learning and memory. Curr Opin Neurobiol. 2006;16:710–715. [Europe PMC free article] [Abstract] [Google Scholar]
- Hasselmo ME, Bower JM. Acetylcholine and memory. Trends Neurosci. 1993;16:218–222. [Abstract] [Google Scholar]
- He J, Deng CY, Chen RZ, Zhu XN, Yu JP. Long-term potentiation induced by nicotine in CA1 region of hippocampal slice is Ca(2+)-dependent. Acta Pharmacol Sin. 2000;21:429–432. [Abstract] [Google Scholar]
- He J, Deng CY, Zhu XN, Yu JP, Chen RZ. Different synaptic mechanisms of long-term potentiation induced by nicotine and tetanic stimulation in hippocampal CA1 region of rats. Acta Pharmacol Sin. 2003;24:398–402. [Abstract] [Google Scholar]
- Hebb DO. The Organization of Behavior: A Neuropsychological Theory. New York: Wiley; 1949. [Google Scholar]
- Heilbronn E, Bartfai T. Muscarinic acetylcholine receptor. Prog Neurobiol. 1978;11:171–188. [Abstract] [Google Scholar]
- Heishman SJ, Taylor RC, Henningfield JE. Nicotine and smoking a review of effects on human performance. Exp Clin Psychopharm. 1994;2:345–395. [Google Scholar]
- Hendricks PS, Ditre JW, Drobes DJ, Brandon TH. The early time course of smoking withdrawal effects. Psychopharmacology (Berl) 2006;187:385–396. [Abstract] [Google Scholar]
- Henriksen EJ, Colgin LL, Barnes CA, Witter MP, Moser MB, Moser EI. Spatial representation along the proximodistal axis of CA1. Neuron. 2010;68:127–137. [Europe PMC free article] [Abstract] [Google Scholar]
- Hernandez-Rabaza V, Barcia JA, Llorens-Martin M, Trejo JL, Canales JJ. Spared place and object-place learning but limited spatial working memory capacity in rats with selective lesions of the dentate gyrus. Brain Res Bull. 2007;72:315–323. [Abstract] [Google Scholar]
- Hernandez-Rabaza V, Hontecillas-Prieto L, Velazquez-Sanchez C, Ferragud A, Perez-Villaba A, Arcusa A, Barcia JA, Trejo JL, Canales JJ. The hippocampal dentate gyrus is essential for generating contextual memories of fear and drug-induced reward. Neurobiol Learn Mem. 2008;90:553–559. [Abstract] [Google Scholar]
- Hodges H, Allen Y, Kershaw T, Lantos PL, Gray JA, Sinden J. Effects of cholinergic-rich neural grafts on radial maze performance of rats after excitotoxic lesions of the forebrain cholinergic projection system--I. Amelioration of cognitive deficits by transplants into cortex and hippocampus but not into basal forebrain. Neuroscience. 1991a;45:587–607. [Abstract] [Google Scholar]
- Hodges H, Allen Y, Sinden J, Mitchell SN, Arendt T, Lantos PL, Gray JA. The effects of cholinergic drugs and cholinergic-rich foetal neural transplants on alcohol-induced deficits in radial maze performance in rats. Behav Brain Res. 1991b;43:7–28. [Abstract] [Google Scholar]
- Holahan MR, Routtenberg A. Lidocaine injections targeting CA3 hippocampus impair long-term spatial memory and prevent learning-induced mossy fiber remodeling. Hippocampus. 2011;21:532–540. [Europe PMC free article] [Abstract] [Google Scholar]
- Hoover DB, Muth EA, Jacobowitz DM. A mapping of the distribution of acetycholine, choline acetyltransferase and acetylcholinesterase in discrete areas of rat brain. Brain Res. 1978;153:295–306. [Abstract] [Google Scholar]
- Huang YY, Kandel ER. Recruitment of long-lasting and protein kinase A-dependent long-term potentiation in the CA1 region of hippocampus requires repeated tetanization. Learn Mem. 1994;1:74–82. [Abstract] [Google Scholar]
- Hudmon KS, Corelli RL, Prokhorov AV. Current approaches to pharmacotherapy for smoking cessation. Ther Adv Respir Dis. 2010;4:35–47. [Abstract] [Google Scholar]
- Huerta PT, Sun LD, Wilson MA, Tonegawa S. Formation of temporal memory requires NMDA receptors within CA1 pyramidal neurons. Neuron. 2000;25:473–480. [Abstract] [Google Scholar]
- Huff NC, Frank M, Wright-Hardesty K, Sprunger D, Matus-Amat P, Higgins E, Rudy JW. Amygdala regulation of immediate-early gene expression in the hippocampus induced by contextual fear conditioning. J Neurosci. 2006;26:1616–1623. [Europe PMC free article] [Abstract] [Google Scholar]
- Hughes JR. Effects of abstinence from tobacco: valid symptoms and time course. Nicotine Tob Res. 2007;9:315–327. [Abstract] [Google Scholar]
- Hughes JR, Gust SW, Skoog K, Keenan RM, Fenwick JW. Symptoms of tobacco withdrawal. A replication and extension. Arch Gen Psychiatry. 1991;48:52–59. [Abstract] [Google Scholar]
- Hughes JR, Higgins ST, Bickel WK. Nicotine withdrawal versus other drug withdrawal syndromes: similarities and dissimilarities. Addiction. 1994;89:1461–1470. [Abstract] [Google Scholar]
- Hughes JR, Keenan RM, Yellin A. Effect of tobacco withdrawal on sustained attention. Addict Behav. 1989;14:577–580. [Abstract] [Google Scholar]
- Hulihan-Giblin BA, Lumpkin MD, Kellar KJ. Acute effects of nicotine on prolactin release in the rat: agonist and antagonist effects of a single injection of nicotine. J Pharmacol Exp Ther. 1990;252:15–20. [Abstract] [Google Scholar]
- Hunsaker MR, Mooy GG, Swift JS, Kesner RP. Dissociations of the medial and lateral perforant path projections into dorsal DG, CA3, and CA1 for spatial and nonspatial (visual object) information processing. Behav Neurosci. 2007;121:742–750. [Abstract] [Google Scholar]
- Ikemoto S, Witkin BM, Morales M. Rewarding injections of the cholinergic agonist carbachol into the ventral tegmental area induce locomotion and c-Fos expression in the retrosplenial area and supramammillary nucleus. Brain Res. 2003;969:78–87. [Abstract] [Google Scholar]
- Impey S, Obrietan K, Wong ST, Poser S, Yano S, Wayman G, Deloulme JC, Chan G, Storm DR. Cross talk between ERK and PKA is required for Ca2+ stimulation of CREB-dependent transcription and ERK nuclear translocation. Neuron. 1998a;21:869–883. [Abstract] [Google Scholar]
- Impey S, Smith DM, Obrietan K, Donahue R, Wade C, Storm DR. Stimulation of cAMP response element (CRE)-mediated transcription during contextual learning. Nat Neurosci. 1998b;1:595–601. [Abstract] [Google Scholar]
- Iniguez SD, Warren BL, Parise EM, Alcantara LF, Schuh B, Maffeo ML, Manojlovic Z, Bolanos-Guzman CA. Nicotine exposure during adolescence induces a depression-like state in adulthood. Neuropsychopharmacology. 2009;34:1609–1624. [Europe PMC free article] [Abstract] [Google Scholar]
- Ishizuka N. Laminar organization of the pyramidal cell layer of the subiculum in the rat. J Comp Neurol. 2001;435:89–110. [Abstract] [Google Scholar]
- Ishizuka N, Weber J, Amaral DG. Organization of intrahippocampal projections originating from CA3 pyramidal cells in the rat. J Comp Neurol. 1990;295:580–623. [Abstract] [Google Scholar]
- Izaki Y, Takita M, Akema T. Specific role of the posterior dorsal hippocampus-prefrontal cortex in short-term working memory. Eur J Neurosci. 2008;27:3029–3034. [Abstract] [Google Scholar]
- Jacobsen LK, Krystal JH, Mencl WE, Westerveld M, Frost SJ, Pugh KR. Effects of smoking and smoking abstinence on cognition in adolescent tobacco smokers. Biol Psychiatry. 2005;57:56–66. [Abstract] [Google Scholar]
- Jacobsen LK, Mencl WE, Constable RT, Westerveld M, Pugh KR. Impact of smoking abstinence on working memory neurocircuitry in adolescent daily tobacco smokers. Psychopharmacology (Berl) 2007;193:557–566. [Abstract] [Google Scholar]
- Jay TM, Witter MP. Distribution of hippocampal CA1 and subicular efferents in the prefrontal cortex of the rat studied by means of anterograde transport of Phaseolus vulgaris-leucoagglutinin. J Comp Neurol. 1991;313:574–586. [Abstract] [Google Scholar]
- Jeltsch H, Bertrand F, Lazarus C, Cassel JC. Cognitive performances and locomotor activity following dentate granule cell damage in rats: role of lesion extent and type of memory tested. Neurobiol Learn Mem. 2001;76:81–105. [Abstract] [Google Scholar]
- Jerman T, Kesner RP, Hunsaker MR. Disconnection analysis of CA3 and DG in mediating encoding but not retrieval in a spatial maze learning task. Learn Mem. 2006;13:458–464. [Europe PMC free article] [Abstract] [Google Scholar]
- Jia Y, Yamazaki Y, Nakauchi S, Ito K, Sumikawa K. Nicotine facilitates long-term potentiation induction in oriens-lacunosum moleculare cells via Ca2+ entry through non-alpha7 nicotinic acetylcholine receptors. Eur J Neurosci. 2010;31:463–476. [Europe PMC free article] [Abstract] [Google Scholar]
- Jia Y, Yamazaki Y, Nakauchi S, Sumikawa K. Alpha2 nicotine receptors function as a molecular switch to continuously excite a subset of interneurons in rat hippocampal circuits. Eur J Neurosci. 2009;29:1588–1603. [Europe PMC free article] [Abstract] [Google Scholar]
- Johnston LD, O’Malley PM, Bachman JG, Schulenberg JE. Monitoring the Future national results on adolescent drug use: Overview of key findings 2011. Ann Arbor: The University of Michigan; 2012. p. 78. [Google Scholar]
- Jorenby DE, Hays JT, Rigotti NA, Azoulay S, Watsky EJ, Williams KE, Billing CB, Gong J, Reeves KR. Efficacy of varenicline, an alpha4beta2 nicotinic acetylcholine receptor partial agonist, vs placebo or sustained-release bupropion for smoking cessation: a randomized controlled trial. Jama. 2006;296:56–63. [Abstract] [Google Scholar]
- Karadsheh MS, Shah MS, Tang X, Macdonald RL, Stitzel JA. Functional characterization of mouse alpha4beta2 nicotinic acetylcholine receptors stably expressed in HEK293T cells. J Neurochem. 2004;91:1138–1150. [Abstract] [Google Scholar]
- Keene CS, Bucci DJ. Damage to the retrosplenial cortex produces specific impairments in spatial working memory. Neurobiol Learn Mem. 2009;91:408–414. [Abstract] [Google Scholar]
- Kelley AE. Functional specificity of ventral striatal compartments in appetitive behaviors. Ann N Y Acad Sci. 1999;877:71–90. [Abstract] [Google Scholar]
- Kelley AE. Memory and addiction: shared neural circuitry and molecular mechanisms. Neuron. 2004;44:161–179. [Abstract] [Google Scholar]
- Kemp G, Morley B, Dwyer D, Bradley RJ. Purification and characterization of nicotinic acetylcholine receptors from muscle. Membr Biochem. 1980;3:229–257. [Abstract] [Google Scholar]
- Kenney JW, Adoff MD, Wilkinson DS, Gould TJ. The effects of acute, chronic, and withdrawal from chronic nicotine on novel and spatial object recognition in male C57BL/6J mice. Psychopharmacology (Berl) 2011;217:353–365. [Europe PMC free article] [Abstract] [Google Scholar]
- Kenney JW, Florian C, Portugal GS, Abel T, Gould TJ. Involvement of hippocampal jun-N terminal kinase pathway in the enhancement of learning and memory by nicotine. Neuropsychopharmacology. 2010;35:483–492. [Europe PMC free article] [Abstract] [Google Scholar]
- Kenney JW, Gould TJ. Nicotine enhances context learning but not context-shock associative learning. Behav Neurosci. 2008;122:1158–1165. [Europe PMC free article] [Abstract] [Google Scholar]
- Kenney JW, Poole RL, Adoff MD, Logue SF, Gould TJ. Learning and Nicotine Interact to Increase CREB Phosphorylation at the jnk1 Promoter in the Hippocampus. PLoS One. 2012a;7:e39939. [Europe PMC free article] [Abstract] [Google Scholar]
- Kenney JW, Raybuck JD, Gould TJ. Nicotinic receptors in the dorsal and ventral hippocampus differentially modulate contextual fear conditioning. Hippocampus. 2012b;22:1681–1690. [Europe PMC free article] [Abstract] [Google Scholar]
- Kesner RP, Hunsaker MR, Warthen MW. The CA3 subregion of the hippocampus is critical for episodic memory processing by means of relational encoding in rats. Behav Neurosci. 2008;122:1217–1225. [Abstract] [Google Scholar]
- Kesner RP, Hunsaker MR, Ziegler W. The role of the dorsal CA1 and ventral CA1 in memory for the temporal order of a sequence of odors. Neurobiol Learn Mem. 2011;93:111–116. [Abstract] [Google Scholar]
- Kikusui T, Tonohiro T, Kaneko T. The allocentric place discrimination task is selectively and highly dependent on the central muscarinic system in rats. Pharmacol Biochem Behav. 2000;65:131–139. [Abstract] [Google Scholar]
- Kim JJ, Fanselow MS. Modality-specific retrograde amnesia of fear. Science. 1992;256:675–677. [Abstract] [Google Scholar]
- Kjelstrup KG, Tuvnes FA, Steffenach HA, Murison R, Moser EI, Moser MB. Reduced fear expression after lesions of the ventral hippocampus. Proc Natl Acad Sci U S A. 2002;99:10825–10830. [Europe PMC free article] [Abstract] [Google Scholar]
- Klann E, Chen SJ, Sweatt JD. Persistent protein kinase activation in the maintenance phase of long-term potentiation. J Biol Chem. 1991;266:24253–24256. [Abstract] [Google Scholar]
- Kleinman KM, Vaughn RL, Christ TS. Effects of cigarette smoking and smoking deprivation on paired-associate learning of high and low meaningful nonsense syllables. Psychol Rep. 1973;32:963–966. [Abstract] [Google Scholar]
- Kota D, Robinson SE, Imad Damaj M. Enhanced nicotine reward in adulthood after exposure to nicotine during early adolescence in mice. Biochem Pharmacol. 2009;78:873–879. [Abstract] [Google Scholar]
- Kratochvil CJ, Vaughan BS, Harrington MJ, Burke WJ. Atomoxetine: a selective noradrenaline reuptake inhibitor for the treatment of attention-deficit/hyperactivity disorder. Expert Opin Pharmacother. 2003;4:1165–1174. [Abstract] [Google Scholar]
- Krug M, Lossner B, Ott T. Anisomycin blocks the late phase of long-term potentiation in the dentate gyrus of freely moving rats. Brain Res Bull. 1984;13:39–42. [Abstract] [Google Scholar]
- Kuryatov A, Luo J, Cooper J, Lindstrom J. Nicotine acts as a pharmacological chaperone to up-regulate human alpha4beta2 acetylcholine receptors. Mol Pharmacol. 2005;68:1839–1851. [Abstract] [Google Scholar]
- Lassalle JM, Bataille T, Halley H. Reversible inactivation of the hippocampal mossy fiber synapses in mice impairs spatial learning, but neither consolidation nor memory retrieval, in the Morris navigation task. Neurobiol Learn Mem. 2000;73:243–257. [Abstract] [Google Scholar]
- Laviola G, Adriani W, Terranova ML, Gerra G. Psychobiologic risk factors and vulnerability to psychostimulants in adolescents and animal models. Ann Ist Super Sanita. 2000;36:47–62. [Abstract] [Google Scholar]
- Lax P, Fucile S, Eusebi F. Ca(2+) permeability of human heteromeric nAChRs expressed by transfection in human cells. Cell Calcium. 2002;32:53–58. [Abstract] [Google Scholar]
- Le Novere N, Corringer PJ, Changeux JP. The diversity of subunit composition in nAChRs: evolutionary origins, physiologic and pharmacologic consequences. J Neurobiol. 2002;53:447–456. [Abstract] [Google Scholar]
- Leach PT, Cordero KA, Gould TJ. The effects of acute nicotine, chronic nicotine, and withdrawal from chronic nicotine on performance of a cued appetitive response. Behav Neurosci. 2013;127:303–310. [Europe PMC free article] [Abstract] [Google Scholar]
- Leach PT, Poplawski SG, Kenney JW, Hoffman B, Liebermann DA, Abel T, Gould TJ. Gadd45b knockout mice exhibit selective deficits in hippocampus-dependent long-term memory. Learn Mem. 2012;19:319–324. [Europe PMC free article] [Abstract] [Google Scholar]
- LeDoux JE, Thompson ME, Iadecola C, Tucker LW, Reis DJ. Local cerebral blood flow increases during auditory and emotional processing in the conscious rat. Science. 1983;221:576–578. [Abstract] [Google Scholar]
- Lee I, Hunsaker MR, Kesner RP. The role of hippocampal subregions in detecting spatial novelty. Behav Neurosci. 2005a;119:145–153. [Abstract] [Google Scholar]
- Lee I, Jerman TS, Kesner RP. Disruption of delayed memory for a sequence of spatial locations following CA1- or CA3-lesions of the dorsal hippocampus. Neurobiol Learn Mem. 2005b;84:138–147. [Abstract] [Google Scholar]
- Lee I, Kesner RP. Differential roles of dorsal hippocampal subregions in spatial working memory with short versus intermediate delay. Behav Neurosci. 2003;117:1044–1053. [Abstract] [Google Scholar]
- Lee I, Kesner RP. Differential contributions of dorsal hippocampal subregions to memory acquisition and retrieval in contextual fear-conditioning. Hippocampus. 2004;14:301–310. [Abstract] [Google Scholar]
- Leonard S, Adams C, Breese CR, Adler LE, Bickford P, Byerley W, Coon H, Griffith JM, Miller C, Myles-Worsley M, Nagamoto HT, Rollins Y, Stevens KE, Waldo M, Freedman R. Nicotinic receptor function in schizophrenia. Schizophr Bull. 1996;22:431–445. [Abstract] [Google Scholar]
- Leonard S, Gault J, Hopkins J, Logel J, Vianzon R, Short M, Drebing C, Berger R, Venn D, Sirota P, Zerbe G, Olincy A, Ross RG, Adler LE, Freedman R. Association of promoter variants in the alpha7 nicotinic acetylcholine receptor subunit gene with an inhibitory deficit found in schizophrenia. Arch Gen Psychiatry. 2002;59:1085–1096. [Abstract] [Google Scholar]
- Lerman C. Helping smokers quit through pharmacogenetics. LDI Issue Brief. 2006;11:1–4. [Abstract] [Google Scholar]
- Levey AI, Kitt CA, Simonds WF, Price DL, Brann MR. Identification and localization of muscarinic acetylcholine receptor proteins in brain with subtype-specific antibodies. J Neurosci. 1991;11:3218–3226. [Europe PMC free article] [Abstract] [Google Scholar]
- Levin ED, Briggs SJ, Christopher NC, Rose JE. Persistence of chronic nicotine-induced cognitive facilitation. Behav Neural Biol. 1992;58:152–158. [Abstract] [Google Scholar]
- Levin ED, Briggs SJ, Christopher NC, Rose JE. Chronic nicotinic stimulation and blockade effects on working memory. Behav Pharmacol. 1993;4:179–182. [Abstract] [Google Scholar]
- Levin ED, McGurk SR, Rose JE, Butcher LL. Reversal of a mecamylamine-induced cognitive deficit with the D2 agonist, LY 171555. Pharmacol Biochem Behav. 1989;33:919–922. [Abstract] [Google Scholar]
- Li JS, Chao YS. Electrolytic lesions of dorsal CA3 impair episodic-like memory in rats. Neurobiol Learn Mem. 2008;89:192–198. [Abstract] [Google Scholar]
- Li SX, Perry KW, Wong DT. Influence of fluoxetine on the ability of bupropion to modulate extracellular dopamine and norepinephrine concentrations in three mesocorticolimbic areas of rats. Neuropharmacology. 2002;42:181–190. [Abstract] [Google Scholar]
- Li XG, Somogyi P, Ylinen A, Buzsaki G. The hippocampal CA3 network: an in vivo intracellular labeling study. J Comp Neurol. 1994;339:181–208. [Abstract] [Google Scholar]
- Li XM, Li CC, Yu SS, Chen JT, Sabapathy K, Ruan DY. JNK1 contributes to metabotropic glutamate receptor-dependent long-term depression and short-term synaptic plasticity in the mice area hippocampal CA1. Eur J Neurosci. 2007;25:391–396. [Abstract] [Google Scholar]
- Liu X, Ramirez S, Pang PT, Puryear CB, Govindarajan A, Deisseroth K, Tonegawa S. Optogenetic stimulation of a hippocampal engram activates fear memory recall. Nature. 2012;484:381–385. [Europe PMC free article] [Abstract] [Google Scholar]
- Logue SF, Owen EH, Rasmussen DL, Wehner JM. Assessment of locomotor activity, acoustic and tactile startle, and prepulse inhibition of startle in inbred mouse strains and F1 hybrids: implications of genetic background for single gene and quantitative trait loci analyses. Neuroscience. 1997;80:1075–1086. [Abstract] [Google Scholar]
- Logue SF, Paylor R, Wehner JM. Hippocampal lesions cause learning deficits in inbred mice in the Morris water maze and conditioned-fear task. Behav Neurosci. 1997;111:104–113. [Abstract] [Google Scholar]
- Lonze BE, Ginty DD. Function and regulation of CREB family transcription factors in the nervous system. Neuron. 2002;35:605–623. [Abstract] [Google Scholar]
- Loughead J, Ray R, Wileyto EP, Ruparel K, Sanborn P, Siegel S, Gur RC, Lerman C. Effects of the alpha4beta2 partial agonist varenicline on brain activity and working memory in abstinent smokers. Biol Psychiatry. 2010;67:715–721. [Abstract] [Google Scholar]
- Loureiro M, Lecourtier L, Engeln M, Lopez J, Cosquer B, Geiger K, Kelche C, Cassel JC, Pereira de Vasconcelos A. The ventral hippocampus is necessary for expressing a spatial memory. Brain Struct Funct. 2012;217:93–106. [Abstract] [Google Scholar]
- Lu YM, Jia Z, Janus C, Henderson JT, Gerlai R, Wojtowicz JM, Roder JC. Mice lacking metabotropic glutamate receptor 5 show impaired learning and reduced CA1 long-term potentiation (LTP) but normal CA3 LTP. J Neurosci. 1997;17:5196–5205. [Europe PMC free article] [Abstract] [Google Scholar]
- Lynch G, Larson J, Kelso S, Barrionuevo G, Schottler F. Intracellular injections of EGTA block induction of hippocampal long-term potentiation. Nature. 1983;305:719–721. [Abstract] [Google Scholar]
- Ma DK, Jang MH, Guo JU, Kitabatake Y, Chang ML, Pow-Anpongkul N, Flavell RA, Lu B, Ming GL, Song H. Neuronal activity-induced Gadd45b promotes epigenetic DNA demethylation and adult neurogenesis. Science. 2009;323:1074–1077. [Europe PMC free article] [Abstract] [Google Scholar]
- MacLean P. Psychosomatic disease and the visceral brain; recent developments bearing on the Papez theory of emotion. Psychosom Med. 1949;11:338–353. [Abstract] [Google Scholar]
- MacLean PD. Some psychiatric implications of physiological studies on frontotemporal portion of limbic system (visceral brain) Electroencephalogr Clin Neurophysiol. 1952;4:407–418. [Abstract] [Google Scholar]
- Maddux JM, Holland PC. Effects of dorsal or ventral medial prefrontal cortical lesions on five-choice serial reaction time performance in rats. Behav Brain Res. 2011;221:63–74. [Europe PMC free article] [Abstract] [Google Scholar]
- Maggio N, Segal M. Striking variations in corticosteroid modulation of long-term potentiation along the septotemporal axis of the hippocampus. J Neurosci. 2007a;27:5757–5765. [Europe PMC free article] [Abstract] [Google Scholar]
- Maggio N, Segal M. Unique regulation of long term potentiation in the rat ventral hippocampus. Hippocampus. 2007b;17:10–25. [Abstract] [Google Scholar]
- Magloczky Z, Acsady L, Freund TF. Principal cells are the postsynaptic targets of supramammillary afferents in the hippocampus of the rat. Hippocampus. 1994;4:322–334. [Abstract] [Google Scholar]
- Mansvelder HD, De Rover M, McGehee DS, Brussaard AB. Cholinergic modulation of dopaminergic reward areas: upstream and downstream targets of nicotine addiction. Eur J Pharmacol. 2003;480:117–123. [Abstract] [Google Scholar]
- Mansvelder HD, van Aerde KI, Couey JJ, Brussaard AB. Nicotinic modulation of neuronal networks: from receptors to cognition. Psychopharmacology (Berl) 2006;184:292–305. [Abstract] [Google Scholar]
- Mao D, Perry DC, Yasuda RP, Wolfe BB, Kellar KJ. The alpha4beta2alpha5 nicotinic cholinergic receptor in rat brain is resistant to up-regulation by nicotine in vivo. J Neurochem. 2008;104:446–456. [Abstract] [Google Scholar]
- Marchi M, Risso F, Viola C, Cavazzani P, Raiteri M. Direct evidence that release-stimulating alpha7* nicotinic cholinergic receptors are localized on human and rat brain glutamatergic axon terminals. J Neurochem. 2002;80:1071–1078. [Abstract] [Google Scholar]
- Maren S, Aharonov G, Fanselow MS. Neurotoxic lesions of the dorsal hippocampus and Pavlovian fear conditioning in rats. Behav Brain Res. 1997;88:261–274. [Abstract] [Google Scholar]
- Maren S, Holt WG. Hippocampus and Pavlovian fear conditioning in rats: muscimol infusions into the ventral, but not dorsal, hippocampus impair the acquisition of conditional freezing to an auditory conditional stimulus. Behav Neurosci. 2004;118:97–110. [Abstract] [Google Scholar]
- Marks MJ. Desensitization and the Regulation of Neuronal Nicotinic Receptors. In: Arneric SP, Brioni JD, editors. Neuronal Nicotinic Receptors: Pharmacology and Therapeutic Opportunities. New York: Wiley-Liss; 1999. pp. 65–80. [Google Scholar]
- Marks MJ, Burch JB, Collins AC. Effects of chronic nicotine infusion on tolerance development and nicotinic receptors. J Pharmacol Exp Ther. 1983;226:817–825. [Abstract] [Google Scholar]
- Marks MJ, Collins AC. Characterization of nicotine binding in mouse brain and comparison with the binding of alpha-bungarotoxin and quinuclidinyl benzilate. Mol Pharmacol. 1982;22:554–564. [Abstract] [Google Scholar]
- Marks MJ, Grady SR, Collins AC. Downregulation of nicotinic receptor function after chronic nicotine infusion. J Pharmacol Exp Ther. 1993;266:1268–1276. [Abstract] [Google Scholar]
- Marks MJ, Stitzel JA, Collins AC. Time course study of the effects of chronic nicotine infusion on drug response and brain receptors. J Pharmacol Exp Ther. 1985;235:619–628. [Abstract] [Google Scholar]
- Marks MJ, Stitzel JA, Romm E, Wehner JM, Collins AC. Nicotinic binding sites in rat and mouse brain: comparison of acetylcholine, nicotine, and alpha-bungarotoxin. Mol Pharmacol. 1986;30:427–436. [Abstract] [Google Scholar]
- Marschner A, Mell T, Wartenburger I, Villringer A, Reischies FM, Heekeren HR. Reward-based decision-making and aging. Brain Res Bull. 2005;67:382–390. [Abstract] [Google Scholar]
- Martin LF, Freedman R. Schizophrenia and the alpha7 nicotinic acetylcholine receptor. Int Rev Neurobiol. 2007;78:225–246. [Abstract] [Google Scholar]
- Mathiasen JR, DiCamillo A. Novel object recognition in the rat: a facile assay for cognitive function. Curr Protoc Pharmacol. 2010;5(5):59. [Abstract] [Google Scholar]
- Matsuyama S, Matsumoto A. Epibatidine induces long-term potentiation (LTP) via activation of alpha4beta2 nicotinic acetylcholine receptors (nAChRs) in vivo in the intact mouse dentate gyrus: both alpha7 and alpha4beta2 nAChRs essential to nicotinic LTP. J Pharmacol Sci. 2003;93:180–187. [Abstract] [Google Scholar]
- Matsuyama S, Matsumoto A, Enomoto T, Nishizaki T. Activation of nicotinic acetylcholine receptors induces long-term potentiation in vivo in the intact mouse dentate gyrus. Eur J Neurosci. 2000;12:3741–3747. [Abstract] [Google Scholar]
- Maviel T, Durkin TP, Menzaghi F, Bontempi B. Sites of neocortical reorganization critical for remote spatial memory. Science. 2004;305:96–99. [Abstract] [Google Scholar]
- Mazzaferro S, Benallegue N, Carbone A, Gasparri F, Vijayan R, Biggin PC, Moroni M, Bermudez I. Additional acetylcholine (ACh) binding site at alpha4/alpha4 interface of (alpha4beta2)2alpha4 nicotinic receptor influences agonist sensitivity. J Biol Chem. 2011;286:31043–31054. [Europe PMC free article] [Abstract] [Google Scholar]
- McClelland JL, McNaughton BL, O’Reilly RC. Why there are complementary learning systems in the hippocampus and neocortex: insights from the successes and failures of connectionist models of learning and memory. Psychol Rev. 1995;102:419–457. [Abstract] [Google Scholar]
- McDonald RJ, Yim TT, Lehmann H, Sparks FT, Zelinski EL, Sutherland RJ, Hong NS. Expression of a conditioned place preference or spatial navigation task following muscimol-induced inactivations of the amygdala or dorsal hippocampus: A double dissociation in the retrograde direction. Brain Res Bull. 2010;83:29–37. [Abstract] [Google Scholar]
- McEchron MD, Bouwmeester H, Tseng W, Weiss C, Disterhoft JF. Hippocampectomy disrupts auditory trace fear conditioning and contextual fear conditioning in the rat. Hippocampus. 1998;8:638–646. [Abstract] [Google Scholar]
- McEown K, Treit D. The role of the dorsal and ventral hippocampus in fear and memory of a shock-probe experience. Brain Res. 2009;1251:185–194. [Abstract] [Google Scholar]
- McEown K, Treit D. Inactivation of the dorsal or ventral hippocampus with muscimol differentially affects fear and memory. Brain Res. 2010;1353:145–151. [Abstract] [Google Scholar]
- McHugh SB, Niewoehner B, Rawlins JN, Bannerman DM. Dorsal hippocampal N-methyl-D-aspartate receptors underlie spatial working memory performance during non-matching to place testing on the T-maze. Behav Brain Res. 2008;186:41–47. [Europe PMC free article] [Abstract] [Google Scholar]
- McHugh TJ, Jones MW, Quinn JJ, Balthasar N, Coppari R, Elmquist JK, Lowell BB, Fanselow MS, Wilson MA, Tonegawa S. Dentate gyrus NMDA receptors mediate rapid pattern separation in the hippocampal network. Science. 2007;317:94–99. [Abstract] [Google Scholar]
- McHugh TJ, Tonegawa S. CA3 NMDA receptors are required for the rapid formation of a salient contextual representation. Hippocampus. 2009;19:1153–1158. [Europe PMC free article] [Abstract] [Google Scholar]
- McKay BE, Placzek AN, Dani JA. Regulation of synaptic transmission and plasticity by neuronal nicotinic acetylcholine receptors. Biochem Pharmacol. 2007;74:1120–1133. [Europe PMC free article] [Abstract] [Google Scholar]
- Meibach RC, Siegel A. Efferent connections of the septal area in the rat: an analysis utilizing retrograde and anterograde transport methods. Brain Res. 1977;119:1–20. [Abstract] [Google Scholar]
- Mendrek A, Monterosso J, Simon SL, Jarvik M, Brody A, Olmstead R, Domier CP, Cohen MS, Ernst M, London ED. Working memory in cigarette smokers: comparison to non-smokers and effects of abstinence. Addict Behav. 2006;31:833–844. [Europe PMC free article] [Abstract] [Google Scholar]
- Merritt PS, Cobb AR, Moissinac L, Hirshman E. Evidence that episodic memory impairment during tobacco abstinence is independent of attentional mechanisms. J Gen Psychol. 2010;137:331–342. [Abstract] [Google Scholar]
- Meyers RA, Zavala AR, Neisewander JL. Dorsal, but not ventral, hippocampal lesions disrupt cocaine place conditioning. Neuroreport. 2003;14:2127–2131. [Abstract] [Google Scholar]
- Middleton RE, Cohen JB. Mapping of the acetylcholine binding site of the nicotinic acetylcholine receptor: [3H]nicotine as an agonist photoaffinity label. Biochemistry. 1991;30:6987–6997. [Abstract] [Google Scholar]
- Mihailescu S, Drucker-Colin R. Nicotine, brain nicotinic receptors, and neuropsychiatric disorders. Arch Med Res. 2000;31:131–144. [Abstract] [Google Scholar]
- Mihalak KB, Carroll FI, Luetje CW. Varenicline is a partial agonist at alpha4beta2 and a full agonist at alpha7 neuronal nicotinic receptors. Mol Pharmacol. 2006;70:801–805. [Abstract] [Google Scholar]
- Misane I, Kruis A, Pieneman AW, Ogren SO, Stiedl O. GABA(A) receptor activation in the CA1 area of the dorsal hippocampus impairs consolidation of conditioned contextual fear in C57BL/6J mice. Behav Brain Res. 2013;238:160–169. [Abstract] [Google Scholar]
- Monmaur P, Collet A, Puma C, Frankel-Kohn L, Sharif A. Relations between acetylcholine release and electrophysiological characteristics of theta rhythm: a microdialysis study in the urethane-anesthetized rat hippocampus. Brain Res Bull. 1997;42:141–146. [Abstract] [Google Scholar]
- Moran PM. Differential effects of scopolamine and mecamylamine on working and reference memory in the rat. Pharmacol Biochem Behav. 1993;45:533–538. [Abstract] [Google Scholar]
- Moroni M, Zwart R, Sher E, Cassels BK, Bermudez I. alpha4beta2 nicotinic receptors with high and low acetylcholine sensitivity: pharmacology, stoichiometry, and sensitivity to long-term exposure to nicotine. Mol Pharmacol. 2006;70:755–768. [Abstract] [Google Scholar]
- Morris RG, Anderson E, Lynch GS, Baudry M. Selective impairment of learning and blockade of long-term potentiation by an N-methyl-D-aspartate receptor antagonist, AP5. Nature. 1986;319:774–776. [Abstract] [Google Scholar]
- Moser EI, Kropff E, Moser MB. Place cells, grid cells, and the brain’s spatial representation system. Annu Rev Neurosci. 2008;31:69–89. [Abstract] [Google Scholar]
- Moser MB, Moser EI. Functional differentiation in the hippocampus. Hippocampus. 1998;8:608–619. [Abstract] [Google Scholar]
- Mosko S, Lynch G, Cotman CW. The distribution of septal projections to the hippocampus of the rat. J Comp Neurol. 1973;152:163–174. [Abstract] [Google Scholar]
- Mugnaini M, Tessari M, Tarter G, Merlo Pich E, Chiamulera C, Bunnemann B. Upregulation of [3H]methyllycaconitine binding sites following continuous infusion of nicotine, without changes of alpha7 or alpha6 subunit mRNA: an autoradiography and in situ hybridization study in rat brain. Eur J Neurosci. 2002;16:1633–1646. [Abstract] [Google Scholar]
- Nader MA, Morgan D, Gage HD, Nader SH, Calhoun TL, Buchheimer N, Ehrenkaufer R, Mach RH. PET imaging of dopamine D2 receptors during chronic cocaine self-administration in monkeys. Nat Neurosci. 2006;9:1050–1056. [Abstract] [Google Scholar]
- Naeve GS, Ramakrishnan M, Kramer R, Hevroni D, Citri Y, Theill LE. Neuritin: a gene induced by neural activity and neurotrophins that promotes neuritogenesis. Proc Natl Acad Sci U S A. 1997;94:2648–2653. [Europe PMC free article] [Abstract] [Google Scholar]
- Nakauchi S, Brennan RJ, Boulter J, Sumikawa K. Nicotine gates long-term potentiation in the hippocampal CA1 region via the activation of alpha2* nicotinic ACh receptors. Eur J Neurosci. 2007;25:2666–2681. [Abstract] [Google Scholar]
- Nakauchi S, Sumikawa K. Endogenously released ACh and exogenous nicotine differentially facilitate long-term potentiation induction in the hippocampal CA1 region of mice. Eur J Neurosci. 2012;35:1381–1395. [Europe PMC free article] [Abstract] [Google Scholar]
- Nakazawa K, Quirk MC, Chitwood RA, Watanabe M, Yeckel MF, Sun LD, Kato A, Carr CA, Johnston D, Wilson MA, Tonegawa S. Requirement for hippocampal CA3 NMDA receptors in associative memory recall. Science. 2002;297:211–218. [Europe PMC free article] [Abstract] [Google Scholar]
- Nakazawa K, Sun LD, Quirk MC, Rondi-Reig L, Wilson MA, Tonegawa S. Hippocampal CA3 NMDA receptors are crucial for memory acquisition of one-time experience. Neuron. 2003;38:305–315. [Abstract] [Google Scholar]
- Nelson ME, Kuryatov A, Choi CH, Zhou Y, Lindstrom J. Alternate stoichiometries of alpha4beta2 nicotinic acetylcholine receptors. Mol Pharmacol. 2003;63:332–341. [Abstract] [Google Scholar]
- Nestler EJ. Common molecular and cellular substrates of addiction and memory. Neurobiol Learn Mem. 2002;78:637–647. [Abstract] [Google Scholar]
- Nestler EJ, Barrot M, DiLeone RJ, Eisch AJ, Gold SJ, Monteggia LM. Neurobiology of depression. Neuron. 2002;34:13–25. [Abstract] [Google Scholar]
- Nguyen PV, Abel T, Kandel ER. Requirement of a critical period of transcription for induction of a late phase of LTP. Science. 1994;265:1104–1107. [Abstract] [Google Scholar]
- Niewoehner B, Single FN, Hvalby O, Jensen V, Meyer zum Alten Borgloh S, Seeburg PH, Rawlins JN, Sprengel R, Bannerman DM. Impaired spatial working memory but spared spatial reference memory following functional loss of NMDA receptors in the dentate gyrus. Eur J Neurosci. 2007;25:837–846. [Europe PMC free article] [Abstract] [Google Scholar]
- O’Keefe J, Dostrovsky J. The hippocampus as a spatial map. Preliminary evidence from unit activity in the freely-moving rat. Brain Res. 1971;34:171–175. [Abstract] [Google Scholar]
- Ogren SO, Schott PA, Kehr J, Yoshitake T, Misane I, Mannstrom P, Sandin J. Modulation of acetylcholine and serotonin transmission by galanin. Relationship to spatial and aversive learning. Ann N Y Acad Sci. 1998;863:342–363. [Abstract] [Google Scholar]
- Olale F, Gerzanich V, Kuryatov A, Wang F, Lindstrom J. Chronic nicotine exposure differentially affects the function of human alpha3, alpha4, and alpha7 neuronal nicotinic receptor subtypes. J Pharmacol Exp Ther. 1997;283:675–683. [Abstract] [Google Scholar]
- Olincy A, Harris JG, Johnson LL, Pender V, Kongs S, Allensworth D, Ellis J, Zerbe GO, Leonard S, Stevens KE, Stevens JO, Martin L, Adler LE, Soti F, Kem WR, Freedman R. Proof-of-concept trial of an alpha7 nicotinic agonist in schizophrenia. Arch Gen Psychiatry. 2006;63:630–638. [Abstract] [Google Scholar]
- Olincy A, Stevens KE. Treating schizophrenia symptoms with an alpha7 nicotinic agonist, from mice to men. Biochem Pharmacol. 2007;74:1192–1201. [Europe PMC free article] [Abstract] [Google Scholar]
- Orr-Urtreger A, Goldner FM, Saeki M, Lorenzo I, Goldberg L, De Biasi M, Dani JA, Patrick JW, Beaudet AL. Mice deficient in the alpha7 neuronal nicotinic acetylcholine receptor lack alpha-bungarotoxin binding sites and hippocampal fast nicotinic currents. J Neurosci. 1997;17:9165–9171. [Europe PMC free article] [Abstract] [Google Scholar]
- Otani S, Marshall CJ, Tate WP, Goddard GV, Abraham WC. Maintenance of long-term potentiation in rat dentate gyrus requires protein synthesis but not messenger RNA synthesis immediately post-tetanization. Neuroscience. 1989;28:519–526. [Abstract] [Google Scholar]
- Owen EH, Logue SF, Rasmussen DL, Wehner JM. Assessment of learning by the Morris water task and fear conditioning in inbred mouse strains and F1 hybrids: implications of genetic background for single gene mutations and quantitative trait loci analyses. Neuroscience. 1997;80:1087–1099. [Abstract] [Google Scholar]
- Papatheodoropoulos C, Kostopoulos G. Decreased ability of rat temporal hippocampal CA1 region to produce long-term potentiation. Neurosci Lett. 2000;279:177–180. [Abstract] [Google Scholar]
- Papez JW. A proposed mechanism of emotion. 1937. J Neuropsychiatry Clin Neurosci. 1937;7:103–112. [Abstract] [Google Scholar]
- Papke RL, Trocme-Thibierge C, Guendisch D, Al Rubaiy SA, Bloom SA. Electrophysiological perspectives on the therapeutic use of nicotinic acetylcholine receptor partial agonists. J Pharmacol Exp Ther. 2011;337:367–379. [Europe PMC free article] [Abstract] [Google Scholar]
- Patterson F, Jepson C, Loughead J, Perkins K, Strasser AA, Siegel S, Frey J, Gur R, Lerman C. Working memory deficits predict short-term smoking resumption following brief abstinence. Drug Alcohol Depend. 2010;106:61–64. [Europe PMC free article] [Abstract] [Google Scholar]
- Patterson F, Jepson C, Strasser AA, Loughead J, Perkins KA, Gur RC, Frey JM, Siegel S, Lerman C. Varenicline improves mood and cognition during smoking abstinence. Biol Psychiatry. 2009;65:144–149. [Europe PMC free article] [Abstract] [Google Scholar]
- Paylor R, Nguyen M, Crawley JN, Patrick J, Beaudet A, Orr-Urtreger A. Alpha7 nicotinic receptor subunits are not necessary for hippocampal-dependent learning or sensorimotor gating: a behavioral characterization of Acra7-deficient mice. Learn Mem. 1998;5:302–316. [Europe PMC free article] [Abstract] [Google Scholar]
- Penfield W, Milner B. Memory deficit produced by bilateral lesions in the hippocampal zone. AMA Arch Neurol Psychiatry. 1958;79:475–497. [Abstract] [Google Scholar]
- Pentkowski NS, Blanchard DC, Lever C, Litvin Y, Blanchard RJ. Effects of lesions to the dorsal and ventral hippocampus on defensive behaviors in rats. Eur J Neurosci. 2006;23:2185–2196. [Abstract] [Google Scholar]
- Penton RE, Quick MW, Lester RA. Short- and long-lasting consequences of in vivo nicotine treatment on hippocampal excitability. J Neurosci. 2011;31:2584–2594. [Europe PMC free article] [Abstract] [Google Scholar]
- Perry DC, Xiao Y, Nguyen HN, Musachio JL, Davila-Garcia MI, Kellar KJ. Measuring nicotinic receptors with characteristics of alpha4beta2, alpha3beta2 and alpha3beta4 subtypes in rat tissues by autoradiography. J Neurochem. 2002;82:468–481. [Abstract] [Google Scholar]
- Perry E, Walker M, Grace J, Perry R. Acetylcholine in mind: a neurotransmitter correlate of consciousness? Trends Neurosci. 1999;22:273–280. [Abstract] [Google Scholar]
- Phillips RG, LeDoux JE. Differential contribution of amygdala and hippocampus to cued and contextual fear conditioning. Behav Neurosci. 1992;106:274–285. [Abstract] [Google Scholar]
- Picciotto MR. Nicotine as a modulator of behavior: beyond the inverted U. Trends Pharmacol Sci. 2003;24:493–499. [Abstract] [Google Scholar]
- Picciotto MR, Caldarone BJ, King SL, Zachariou V. Nicotinic receptors in the brain. Links between molecular biology and behavior. Neuropsychopharmacology. 2000;22:451–465. [Abstract] [Google Scholar]
- Pikkarainen M, Ronkko S, Savander V, Insausti R, Pitkanen A. Projections from the lateral, basal, and accessory basal nuclei of the amygdala to the hippocampal formation in rat. J Comp Neurol. 1999;403:229–260. [Abstract] [Google Scholar]
- Place R, Lykken C, Beer Z, Suh J, McHugh TJ, Tonegawa S, Eichenbaum H, Sauvage MM. NMDA signaling in CA1 mediates selectively the spatial component of episodic memory. Learn Mem. 2012;19:164–169. [Europe PMC free article] [Abstract] [Google Scholar]
- Platenik J, Kuramoto N, Yoneda Y. Molecular mechanisms associated with long-term consolidation of the NMDA signals. Life Sci. 2000;67:335–364. [Abstract] [Google Scholar]
- Polesskaya OO, Fryxell KJ, Merchant AD, Locklear LL, Ker KF, McDonald CG, Eppolito AK, Smith LN, Wheeler TL, Smith RF. Nicotine causes age-dependent changes in gene expression in the adolescent female rat brain. Neurotoxicol Teratol. 2007;29:126–140. [Abstract] [Google Scholar]
- Portugal GS, Gould TJ. Bupropion dose-dependently reverses nicotine withdrawal deficits in contextual fear conditioning. Pharmacol Biochem Behav. 2007;88:179–187. [Europe PMC free article] [Abstract] [Google Scholar]
- Portugal GS, Gould TJ. Genetic variability in nicotinic acetylcholine receptors and nicotine addiction: converging evidence from human and animal research. Behav Brain Res. 2008;193:1–16. [Europe PMC free article] [Abstract] [Google Scholar]
- Portugal GS, Gould TJ. Nicotine withdrawal disrupts new contextual learning. Pharmacol Biochem Behav. 2009;92:117–123. [Europe PMC free article] [Abstract] [Google Scholar]
- Portugal GS, Kenney JW, Gould TJ. Beta2 subunit containing acetylcholine receptors mediate nicotine withdrawal deficits in the acquisition of contextual fear conditioning. Neurobiol Learn Mem. 2008;89:106–113. [Europe PMC free article] [Abstract] [Google Scholar]
- Portugal GS, Wilkinson DS, Kenney JW, Sullivan C, Gould TJ. Strain-dependent effects of acute, chronic, and withdrawal from chronic nicotine on fear conditioning. Behav Genet. 2012a;42:133–150. [Europe PMC free article] [Abstract] [Google Scholar]
- Portugal GS, Wilkinson DS, Turner JR, Blendy JA, Gould TJ. Developmental effects of acute, chronic, and withdrawal from chronic nicotine on fear conditioning. Neurobiol Learn Mem. 2012b;97:482–494. [Europe PMC free article] [Abstract] [Google Scholar]
- Poser S, Storm DR. Role of Ca2+-stimulated adenylyl cyclases in LTP and memory formation. Int J Dev Neurosci. 2001;19:387–394. [Abstract] [Google Scholar]
- Radcliffe KA, Fisher JL, Gray R, Dani JA. Nicotinic modulation of glutamate and GABA synaptic transmission of hippocampal neurons. Ann N Y Acad Sci. 1999;868:591–610. [Abstract] [Google Scholar]
- Ragozzino D, Barabino B, Fucile S, Eusebi F. Ca2+ permeability of mouse and chick nicotinic acetylcholine receptors expressed in transiently transfected human cells. J Physiol. 1998;507(Pt 3):749–757. [Abstract] [Google Scholar]
- Raisman G. The connexions of the septum. Brain. 1966;89:317–348. [Abstract] [Google Scholar]
- Raisman G, Cowan WM, Powell TP. An experimental analysis of the efferent projection of the hippocampus. Brain. 1966;89:83–108. [Abstract] [Google Scholar]
- Ramóny Cajal S. Histologie du Système Nerveux de l’Homme et des Vertébrés. A. Maloine; 1911. [Google Scholar]
- Raybuck JD, Gould TJ. Extracellular signal-regulated kinase 1/2 involvement in the enhancement of contextual fear conditioning by nicotine. Behav Neurosci. 2007;121:1119–1124. [Europe PMC free article] [Abstract] [Google Scholar]
- Raybuck JD, Gould TJ. Nicotine withdrawal-induced deficits in trace fear conditioning in C57BL/6 mice--a role for high-affinity beta2 subunit-containing nicotinic acetylcholine receptors. Eur J Neurosci. 2009;29:377–387. [Europe PMC free article] [Abstract] [Google Scholar]
- Raybuck JD, Portugal GS, Lerman C, Gould TJ. Varenicline ameliorates nicotine withdrawal-induced learning deficits in C57BL/6 mice. Behav Neurosci. 2008;122:1166–1171. [Europe PMC free article] [Abstract] [Google Scholar]
- Remondes M, Schuman EM. Role for a cortical input to hippocampal area CA1 in the consolidation of a long-term memory. Nature. 2004;431:699–703. [Abstract] [Google Scholar]
- Rezvani AH, Levin ED. Cognitive effects of nicotine. Biol Psychiatry. 2001;49:258–267. [Abstract] [Google Scholar]
- Rhodes JD, Hawk LW, Jr, Ashare RL, Schlienz NJ, Mahoney MC. The effects of varenicline on attention and inhibitory control among treatment-seeking smokers. Psychopharmacology (Berl) 2012;223:131–138. [Abstract] [Google Scholar]
- Ribak CE, Seress L, Amaral DG. The development, ultrastructure and synaptic connections of the mossy cells of the dentate gyrus. J Neurocytol. 1985;14:835–857. [Abstract] [Google Scholar]
- Ridley RM, Timothy CJ, Maclean CJ, Baker HF. Conditional learning and memory impairments following neurotoxic lesion of the CA1 field of the hippocampus. Neuroscience. 1995;67:263–275. [Abstract] [Google Scholar]
- Riekkinen M, Riekkinen P., Jr Effects of THA and physostigmine on spatial navigation and avoidance performance in mecamylamine and PCPA-treated rats. Exp Neurol. 1994;125:111–118. [Abstract] [Google Scholar]
- Risinger FO, Oakes RA. Nicotine-induced conditioned place preference and conditioned place aversion in mice. Pharmacol Biochem Behav. 1995;51:457–461. [Abstract] [Google Scholar]
- Rodriguez P, Levy WB. A model of hippocampal activity in trace conditioning: where’s the trace? Behav Neurosci. 2001;115:1224–1238. [Abstract] [Google Scholar]
- Rogers AT, Wonnacott S. Nicotine-induced upregulation of alpha bungarotoxin (alpha Bgt) binding sites in cultured rat hippocampal neurons. Biochem Soc Trans. 1995;23:48S. [Abstract] [Google Scholar]
- Rollema H, Chambers LK, Coe JW, Glowa J, Hurst RS, Lebel LA, Lu Y, Mansbach RS, Mather RJ, Rovetti CC, Sands SB, Schaeffer E, Schulz DW, Tingley FD, 3rd, Williams KE. Pharmacological profile of the alpha4beta2 nicotinic acetylcholine receptor partial agonist varenicline, an effective smoking cessation aid. Neuropharmacology. 2007;52:985–994. [Abstract] [Google Scholar]
- Roozendaal B, Phillips RG, Power AE, Brooke SM, Sapolsky RM, McGaugh JL. Memory retrieval impairment induced by hippocampal CA3 lesions is blocked by adrenocortical suppression. Nat Neurosci. 2001;4:1169–1171. [Abstract] [Google Scholar]
- Rosato-Siri M, Cattaneo A, Cherubini E. Nicotine-induced enhancement of synaptic plasticity at CA3-CA1 synapses requires GABAergic interneurons in adult anti-NGF mice. J Physiol. 2006;576:361–377. [Abstract] [Google Scholar]
- Rose EJ, Ross TJ, Salmeron BJ, Lee M, Shakleya DM, Huestis M, Stein EA. Chronic exposure to nicotine is associated with reduced reward-related activity in the striatum but not the midbrain. Biol Psychiatry. 2012;71:206–213. [Europe PMC free article] [Abstract] [Google Scholar]
- Royer S, Sirota A, Patel J, Buzsaki G. Distinct representations and theta dynamics in dorsal and ventral hippocampus. J Neurosci. 2010;30:1777–1787. [Europe PMC free article] [Abstract] [Google Scholar]
- Rubinstein ML, Luks TL, Moscicki AB, Dryden W, Rait MA, Simpson GV. Smoking-related cue-induced brain activation in adolescent light smokers. J Adolesc Health. 2011;48:7–12. [Europe PMC free article] [Abstract] [Google Scholar]
- Rudy JW, Sutherland RJ. The hippocampal formation is necessary for rats to learn and remember configural discriminations. Behav Brain Res. 1989;34:97–109. [Abstract] [Google Scholar]
- Rukstalis M, Jepson C, Patterson F, Lerman C. Increases in hyperactive-impulsive symptoms predict relapse among smokers in nicotine replacement therapy. J Subst Abuse Treat. 2005;28:297–304. [Abstract] [Google Scholar]
- Ruth RE, Collier TJ, Routtenberg A. Topography between the entorhinal cortex and the dentate septotemporal axis in rats: I. Medial and intermediate entorhinal projecting cells. J Comp Neurol. 1982;209:69–78. [Abstract] [Google Scholar]
- Ruth RE, Collier TJ, Routtenberg A. Topographical relationship between the entorhinal cortex and the septotemporal axis of the dentate gyrus in rats: II. Cells projecting from lateral entorhinal subdivisions. J Comp Neurol. 1988;270:506–516. [Abstract] [Google Scholar]
- Sakurai S, Yu L, Tan SE. Roles of hippocampal N-methyl-D-aspartate receptors and calcium/calmodulin-dependent protein kinase II in amphetamine-produced conditioned place preference in rats. Behav Pharmacol. 2007;18:497–506. [Abstract] [Google Scholar]
- Samochocki M, Zerlin M, Jostock R, Groot Kormelink PJ, Luyten WH, Albuquerque EX, Maelicke A. Galantamine is an allosterically potentiating ligand of the human alpha4/beta2 nAChR. Acta Neurol Scand Suppl. 2000;176:68–73. [Abstract] [Google Scholar]
- Sananbenesi F, Fischer A, Schrick C, Spiess J, Radulovic J. Phosphorylation of hippocampal Erk-1/2, Elk-1, and p90-Rsk-1 during contextual fear conditioning: interactions between Erk-1/2 and Elk-1. Mol Cell Neurosci. 2002;21:463–476. [Abstract] [Google Scholar]
- Sannino S, Russo F, Torromino G, Pendolino V, Calabresi P, De Leonibus E. Role of the dorsal hippocampus in object memory load. Learn Mem. 2012;19:211–218. [Abstract] [Google Scholar]
- Sarter M, Bruno JP. Cognitive functions of cortical acetylcholine: toward a unifying hypothesis. Brain Res Brain Res Rev. 1997;23:28–46. [Abstract] [Google Scholar]
- Sarter M, Markowitsch HJ. Involvement of the amygdala in learning and memory: a critical review, with emphasis on anatomical relations. Behav Neurosci. 1985;99:342–380. [Abstract] [Google Scholar]
- Schneider T, Bizarro L, Asherson PJ, Stolerman IP. Hyperactivity, increased nicotine consumption and impaired performance in the five-choice serial reaction time task in adolescent rats prenatally exposed to nicotine. Psychopharmacology (Berl) 2012;223:401–415. [Europe PMC free article] [Abstract] [Google Scholar]
- Schroeder JP, Wingard JC, Packard MG. Post-training reversible inactivation of hippocampus reveals interference between memory systems. Hippocampus. 2002;12:280–284. [Abstract] [Google Scholar]
- Schwartz RD, Kellar KJ. Nicotinic cholinergic receptor binding sites in the brain: regulation in vivo. Science. 1983;220:214–216. [Abstract] [Google Scholar]
- Scoville WB, Milner B. Loss of recent memory after bilateral hippocampal lesions. J Neurol Neurosurg Psychiatry. 1957;20:11–21. [Europe PMC free article] [Abstract] [Google Scholar]
- Seguela P, Wadiche J, Dineley-Miller K, Dani JA, Patrick JW. Molecular cloning, functional properties, and distribution of rat brain alpha 7: a nicotinic cation channel highly permeable to calcium. J Neurosci. 1993;13:596–604. [Europe PMC free article] [Abstract] [Google Scholar]
- Semenova S, Stolerman IP, Markou A. Chronic nicotine administration improves attention while nicotine withdrawal induces performance deficits in the 5-choice serial reaction time task in rats. Pharmacol Biochem Behav. 2007;87:360–368. [Europe PMC free article] [Abstract] [Google Scholar]
- Sershen H, Reith ME, Lajtha A, Gennaro J., Jr Noncholinergic, saturable binding of (+/−)-[3H]nicotine to mouse brain. J Recept Res. 1981;2:1–15. [Abstract] [Google Scholar]
- Sharifzadeh M, Tavasoli M, Naghdi N, Ghanbari A, Amini M, Roghani A. Post-training intrahippocampal infusion of nicotine prevents spatial memory retention deficits induced by the cyclo-oxygenase-2-specific inhibitor celecoxib in rats. J Neurochem. 2005;95:1078–1090. [Abstract] [Google Scholar]
- Sharp BM, Beyer HS. Rapid desensitization of the acute stimulatory effects of nicotine on rat plasma adrenocorticotropin and prolactin. J Pharmacol Exp Ther. 1986;238:486–491. [Abstract] [Google Scholar]
- Shearman E, Rossi S, Sershen H, Hashim A, Lajtha A. Locally administered low nicotine-induced neurotransmitter changes in areas of cognitive function. Neurochem Res. 2005;30:1055–1066. [Abstract] [Google Scholar]
- Shen F, Meredith GE, Napier TC. Amphetamine-induced place preference and conditioned motor sensitization requires activation of tyrosine kinase receptors in the hippocampus. J Neurosci. 2006;26:11041–11051. [Europe PMC free article] [Abstract] [Google Scholar]
- Sherrington CS. The integrative action of the nervous system. New Haven: Yale University Press; 1906. [Google Scholar]
- Shoaib M, Bizarro L. Deficits in a sustained attention task following nicotine withdrawal in rats. Psychopharmacology (Berl) 2005;178:211–222. [Abstract] [Google Scholar]
- Shram MJ, Le AD. Adolescent male Wistar rats are more responsive than adult rats to the conditioned rewarding effects of intravenously administered nicotine in the place conditioning procedure. Behav Brain Res. 2010;206:240–244. [Abstract] [Google Scholar]
- Sierra-Mercado D, Padilla-Coreano N, Quirk GJ. Dissociable roles of prelimbic and infralimbic cortices, ventral hippocampus, and basolateral amygdala in the expression and extinction of conditioned fear. Neuropsychopharmacology. 2011;36:529–538. [Europe PMC free article] [Abstract] [Google Scholar]
- Silva AJ, Kogan JH, Frankland PW, Kida S. CREB and memory. Annu Rev Neurosci. 1998;21:127–148. [Abstract] [Google Scholar]
- Simons SB, Caruana DA, Zhao M, Dudek SM. Caffeine-induced synaptic potentiation in hippocampal CA2 neurons. Nat Neurosci. 2011;15:23–25. [Europe PMC free article] [Abstract] [Google Scholar]
- Slemmer JE, Martin BR, Damaj MI. Bupropion is a nicotinic antagonist. J Pharmacol Exp Ther. 2000;295:321–327. [Abstract] [Google Scholar]
- Slotkin TA, Bodwell BE, Ryde IT, Seidler FJ. Adolescent nicotine treatment changes the response of acetylcholine systems to subsequent nicotine administration in adulthood. Brain Res Bull. 2008;76:152–165. [Abstract] [Google Scholar]
- Smith DM, Barredo J, Mizumori SJ. Complimentary roles of the hippocampus and retrosplenial cortex in behavioral context discrimination. Hippocampus. 2012;22:1121–1133. [Europe PMC free article] [Abstract] [Google Scholar]
- Snyder FR, Davis FC, Henningfield JE. The tobacco withdrawal syndrome: performance decrements assessed on a computerized test battery. Drug Alcohol Depend. 1989;23:259–266. [Abstract] [Google Scholar]
- Socci DJ, Sanberg PR, Arendash GW. Nicotine enhances Morris water maze performance of young and aged rats. Neurobiol Aging. 1995;16:857–860. [Abstract] [Google Scholar]
- Spear LP. The behavioral neuroscience of adolescence. New York: W. W. Norton & Company, Inc; 2010. [Google Scholar]
- Spencer DG, Jr, Horvath E, Traber J. Direct autoradiographic determination of M1 and M2 muscarinic acetylcholine receptor distribution in the rat brain: relation to cholinergic nuclei and projections. Brain Res. 1986;380:59–68. [Abstract] [Google Scholar]
- Squire LR. Memory and the hippocampus: a synthesis from findings with rats, monkeys, and humans. Psychol Rev. 1992;99:195–231. [Abstract] [Google Scholar]
- Squire LR, Alvarez P. Retrograde amnesia and memory consolidation: a neurobiological perspective. Curr Opin Neurobiol. 1995;5:169–177. [Abstract] [Google Scholar]
- Staley JK, Krishnan-Sarin S, Cosgrove KP, Krantzler E, Frohlich E, Perry E, Dubin JA, Estok K, Brenner E, Baldwin RM, Tamagnan GD, Seibyl JP, Jatlow P, Picciotto MR, London ED, O’Malley S, van Dyck CH. Human tobacco smokers in early abstinence have higher levels of beta2* nicotinic acetylcholine receptors than nonsmokers. J Neurosci. 2006;26:8707–8714. [Europe PMC free article] [Abstract] [Google Scholar]
- Steffenach HA, Sloviter RS, Moser EI, Moser MB. Impaired retention of spatial memory after transection of longitudinally oriented axons of hippocampal CA3 pyramidal cells. Proc Natl Acad Sci U S A. 2002;99:3194–3198. [Europe PMC free article] [Abstract] [Google Scholar]
- Steffenach HA, Witter M, Moser MB, Moser EI. Spatial memory in the rat requires the dorsolateral band of the entorhinal cortex. Neuron. 2005;45:301–313. [Abstract] [Google Scholar]
- Stevens R, Cowey A. Effects of dorsal and ventral hippocampal lesions on spontaneous alternation, learned alternation and probability learning in rats. Brain Res. 1973;52:203–224. [Abstract] [Google Scholar]
- Strekalova T, Zorner B, Zacher C, Sadovska G, Herdegen T, Gass P. Memory retrieval after contextual fear conditioning induces c-Fos and JunB expression in CA1 hippocampus. Genes Brain Behav. 2003;2:3–10. [Abstract] [Google Scholar]
- Stubley-Weatherly L, Harding JW, Wright JW. Effects of discrete kainic acid-induced hippocampal lesions on spatial and contextual learning and memory in rats. Brain Res. 1996;716:29–38. [Abstract] [Google Scholar]
- Stupien G, Florian C, Roullet P. Involvement of the hippocampal CA3-region in acquisition and in memory consolidation of spatial but not in object information in mice. Neurobiol Learn Mem. 2003;80:32–41. [Abstract] [Google Scholar]
- Swanson LW. A direct projection from Ammon’s horn to prefrontal cortex in the rat. Brain Res. 1981;217:150–154. [Abstract] [Google Scholar]
- Swanson LW, Cowan WM. Hippocampo-hypothalamic connections: origin in subicular cortex, not ammon’s horn. Science. 1975;189:303–304. [Abstract] [Google Scholar]
- Swanson LW, Kohler C. Anatomical evidence for direct projections from the entorhinal area to the entire cortical mantle in the rat. J Neurosci. 1986;6:3010–3023. [Europe PMC free article] [Abstract] [Google Scholar]
- Takehara K, Kawahara S, Takatsuki K, Kirino Y. Time-limited role of the hippocampus in the memory for trace eyeblink conditioning in mice. Brain Res. 2002;951:183–190. [Abstract] [Google Scholar]
- Taly A, Corringer PJ, Guedin D, Lestage P, Changeux JP. Nicotinic receptors: allosteric transitions and therapeutic targets in the nervous system. Nat Rev Drug Discov. 2009;8:733–750. [Abstract] [Google Scholar]
- Tamai KT, Monaco L, Nantel F, Zazopoulos E, Sassone-Corsi P. Coupling signalling pathways to transcriptional control: nuclear factors responsive to cAMP. Recent Prog Horm Res. 1997;52:121–139. discussion 139–140. [Abstract] [Google Scholar]
- Tang J, Dani JA. Dopamine enables in vivo synaptic plasticity associated with the addictive drug nicotine. Neuron. 2009;63:673–682. [Europe PMC free article] [Abstract] [Google Scholar]
- Tani Y, Saito K, Imoto M, Ohno T. Pharmacological characterization of nicotinic receptor-mediated acetylcholine release in rat brain--an in vivo microdialysis study. Eur J Pharmacol. 1998;351:181–188. [Abstract] [Google Scholar]
- Tarantino LM, Eisener-Dorman AF. Forward Genetic Approaches to Understanding Complex Behaviors. Curr Top Behav Neurosci 2012 [Europe PMC free article] [Abstract] [Google Scholar]
- Tarantino LM, Gould TJ, Druhan JP, Bucan M. Behavior and mutagenesis screens: the importance of baseline analysis of inbred strains. Mamm Genome. 2000;11:555–564. [Abstract] [Google Scholar]
- Tiffany ST. Cognitive concepts of craving. Alcohol Res Health. 1999;23:215–224. [Europe PMC free article] [Abstract] [Google Scholar]
- Tonegawa S, Tsien JZ, McHugh TJ, Huerta P, Blum KI, Wilson MA. Hippocampal CA1-region-restricted knockout of NMDAR1 gene disrupts synaptic plasticity, place fields, and spatial learning. Cold Spring Harb Symp Quant Biol. 1996;61:225–238. [Abstract] [Google Scholar]
- Torres OV, Tejeda HA, Natividad LA, O’Dell LE. Enhanced vulnerability to the rewarding effects of nicotine during the adolescent period of development. Pharmacol Biochem Behav. 2008;90:658–663. [Europe PMC free article] [Abstract] [Google Scholar]
- Trivedi MA, Coover GD. Lesions of the ventral hippocampus, but not the dorsal hippocampus, impair conditioned fear expression and inhibitory avoidance on the elevated T-maze. Neurobiol Learn Mem. 2004;81:172–184. [Abstract] [Google Scholar]
- Tsien JZ, Huerta PT, Tonegawa S. The essential role of hippocampal CA1 NMDA receptor-dependent synaptic plasticity in spatial memory. Cell. 1996;87:1327–1338. [Abstract] [Google Scholar]
- Tucci SA, Genn RF, File SE. Methyllycaconitine (MLA) blocks the nicotine evoked anxiogenic effect and 5-HT release in the dorsal hippocampus: possible role of alpha7 receptors. Neuropharmacology. 2003;44:367–373. [Abstract] [Google Scholar]
- Tulving E. Episodic and Semantic Memory. In: Tulving E, editor. Organization of memory. 1972. [Google Scholar]
- Tzavara ET, Bymaster FP, Overshiner CD, Davis RJ, Perry KW, Wolff M, McKinzie DL, Witkin JM, Nomikos GG. Procholinergic and memory enhancing properties of the selective norepinephrine uptake inhibitor atomoxetine. Mol Psychiatry. 2006;11:187–195. [Abstract] [Google Scholar]
- USDHHS. A Report of the Surgeon General. US Department of Health and Human Services; Maryland: 1988. The health consequences of smoking: nicotine addiction. [Google Scholar]
- USDHHS. 2001 National Household Survey on Drug Abuse: Trends in Initiation of Substance Abuse. Rockville MD: U.S. Department of Health and Human Services, Substance Abuse and Mental Health Services Administration; 2003. [Google Scholar]
- Vago DR, Kesner RP. Cholinergic modulation of Pavlovian fear conditioning in rats: differential effects of intrahippocampal infusion of mecamylamine and methyllycaconitine. Neurobiol Learn Mem. 2007;87:441–449. [Europe PMC free article] [Abstract] [Google Scholar]
- Valentino RJ, Dingledine R. Presynaptic inhibitory effect of acetylcholine in the hippocampus. J Neurosci. 1981;1:784–792. [Europe PMC free article] [Abstract] [Google Scholar]
- van Groen T, Miettinen P, Kadish I. The entorhinal cortex of the mouse: organization of the projection to the hippocampal formation. Hippocampus. 2003;13:133–149. [Abstract] [Google Scholar]
- Vann SD, Aggleton JP. Extensive cytotoxic lesions of the rat retrosplenial cortex reveal consistent deficits on tasks that tax allocentric spatial memory. Behav Neurosci. 2002;116:85–94. [Abstract] [Google Scholar]
- Volkow ND, Fowler JS, Wolf AP, Schlyer D, Shiue CY, Alpert R, Dewey SL, Logan J, Bendriem B, Christman D, et al. Effects of chronic cocaine abuse on postsynaptic dopamine receptors. Am J Psychiatry. 1990;147:719–724. [Abstract] [Google Scholar]
- Wada E, Wada K, Boulter J, Deneris E, Heinemann S, Patrick J, Swanson LW. Distribution of alpha 2, alpha 3, alpha 4, and beta 2 neuronal nicotinic receptor subunit mRNAs in the central nervous system: a hybridization histochemical study in the rat. J Comp Neurol. 1989;284:314–335. [Abstract] [Google Scholar]
- Walters CL, Cleck JN, Kuo YC, Blendy JA. Mu-opioid receptor and CREB activation are required for nicotine reward. Neuron. 2005;46:933–943. [Abstract] [Google Scholar]
- Wang J, Chen YB, Zhu XN, Chen RZ. Activation of p42/44 mitogen-activated protein kinase pathway in long-term potentiation induced by nicotine in hippocampal CA1 region in rats. Acta Pharmacol Sin. 2001;22:685–690. [Abstract] [Google Scholar]
- Wang SH, Finnie PS, Hardt O, Nader K. Dorsal hippocampus is necessary for novel learning but sufficient for subsequent similar learning. Hippocampus. 2012;22:2157–2170. [Abstract] [Google Scholar]
- Wehner JM, Keller JJ, Keller AB, Picciotto MR, Paylor R, Booker TK, Beaudet A, Heinemann SF, Balogh SA. Role of neuronal nicotinic receptors in the effects of nicotine and ethanol on contextual fear conditioning. Neuroscience. 2004;129:11–24. [Abstract] [Google Scholar]
- Welsby P, Rowan M, Anwyl R. Nicotinic receptor-mediated enhancement of long-term potentiation involves activation of metabotropic glutamate receptors and ryanodine-sensitive calcium stores in the dentate gyrus. Eur J Neurosci. 2006;24:3109–3118. [Abstract] [Google Scholar]
- Welsby PJ, Rowan MJ, Anwyl R. Intracellular mechanisms underlying the nicotinic enhancement of LTP in the rat dentate gyrus. Eur J Neurosci. 2009;29:65–75. [Abstract] [Google Scholar]
- Werling LL, McMahon PN, Cox BM. Selective changes in mu opioid receptor properties induced by chronic morphine exposure. Proc Natl Acad Sci U S A. 1989;86:6393–6397. [Europe PMC free article] [Abstract] [Google Scholar]
- Wiegert JS, Hofmann F, Bading H, Bengtson CP. A transcription-dependent increase in miniature EPSC frequency accompanies late-phase plasticity in cultured hippocampal neurons. BMC Neurosci. 2009;10:124. [Europe PMC free article] [Abstract] [Google Scholar]
- Wilkie GI, Hutson P, Sullivan JP, Wonnacott S. Pharmacological characterization of a nicotinic autoreceptor in rat hippocampal synaptosomes. Neurochem Res. 1996;21:1141–1148. [Abstract] [Google Scholar]
- Wilkinson DS, Gould TJ. The effects of galantamine on nicotine withdrawal-induced deficits in contextual fear conditioning in C57BL/6 mice. Behav Brain Res. 2011;223:53–57. [Europe PMC free article] [Abstract] [Google Scholar]
- Wilkinson DS, Gould TJ. Withdrawal from chronic nicotine and subsequent sensitivity to nicotine challenge on contextual learning. Behav Brain Res. 2013;250:58–61. [Europe PMC free article] [Abstract] [Google Scholar]
- Wilkinson DS, Turner JR, Blendy JA, Gould TJ. Genetic background influences the effects of withdrawal from chronic nicotine on learning and high-affinity nicotinic acetylcholine receptor binding in the dorsal and ventral hippocampus. Psychopharmacology (Berl) 2012 [Europe PMC free article] [Abstract] [Google Scholar]
- Wilkinson DS, Yildirim E, Poole RL, Leach PT, Gould TJ. Society for Research on Nicotine and Tobacco. Houston, TX: SRNT; 2012. Nicotine Enhances Long-Term Memory Through a PKA and Protein Synthesis-Dependent Mechanism. [Google Scholar]
- Wilkinson JL, Bevins RA. Intravenous nicotine conditions a place preference in rats using an unbiased design. Pharmacol Biochem Behav. 2008;88:256–264. [Europe PMC free article] [Abstract] [Google Scholar]
- Winters BD, Forwood SE, Cowell RA, Saksida LM, Bussey TJ. Double dissociation between the effects of peri-postrhinal cortex and hippocampal lesions on tests of object recognition and spatial memory: heterogeneity of function within the temporal lobe. J Neurosci. 2004;24:5901–5908. [Europe PMC free article] [Abstract] [Google Scholar]
- Witter MP, Groenewegen HJ. The subiculum: cytoarchitectonically a simple structure, but hodologically complex. Prog Brain Res. 1990;83:47–58. [Abstract] [Google Scholar]
- Witter MP, Groenewegen HJ, Lopes da Silva FH, Lohman AH. Functional organization of the extrinsic and intrinsic circuitry of the parahippocampal region. Prog Neurobiol. 1989;33:161–253. [Abstract] [Google Scholar]
- Witter MP, Ostendorf RH, Groenewegen HJ. Heterogeneity in the Dorsal Subiculum of the Rat. Distinct Neuronal Zones Project to Different Cortical and Subcortical Targets. Eur J Neurosci. 1990;2:718–725. [Abstract] [Google Scholar]
- Wong ST, Athos J, Figueroa XA, Pineda VV, Schaefer ML, Chavkin CC, Muglia LJ, Storm DR. Calcium-stimulated adenylyl cyclase activity is critical for hippocampus-dependent long-term memory and late phase LTP. Neuron. 1999;23:787–798. [Abstract] [Google Scholar]
- Wonnacott S. alpha-Bungarotoxin binds to low-affinity nicotine binding sites in rat brain. J Neurochem. 1986;47:1706–1712. [Abstract] [Google Scholar]
- Woolf NJ, Butcher LL. Cholinergic neurons in the caudate-putamen complex proper are intrinsically organized: a combined Evans blue and acetylcholinesterase analysis. Brain Res Bull. 1981;7:487–507. [Abstract] [Google Scholar]
- Woolf NJ, Butcher LL. Cholinergic systems in the rat brain: III. Projections from the pontomesencephalic tegmentum to the thalamus, tectum, basal ganglia, and basal forebrain. Brain Res Bull. 1986;16:603–637. [Abstract] [Google Scholar]
- Woolf NJ, Eckenstein F, Butcher LL. Cholinergic projections from the basal forebrain to the frontal cortex: a combined fluorescent tracer and immunohistochemical analysis in the rat. Neurosci Lett. 1983;40:93–98. [Abstract] [Google Scholar]
- Woolf NJ, Hernit MC, Butcher LL. Cholinergic and non-cholinergic projections from the rat basal forebrain revealed by combined choline acetyltransferase and Phaseolus vulgaris leucoagglutinin immunohistochemistry. Neurosci Lett. 1986;66:281–286. [Abstract] [Google Scholar]
- Wu K, Leung LS. Monosynaptic activation of CA3 by the medial perforant path. Brain Res. 1998;797:35–41. [Abstract] [Google Scholar]
- Xavier GF, Oliveira-Filho FJ, Santos AM. Dentate gyrus-selective colchicine lesion and disruption of performance in spatial tasks: difficulties in “place strategy” because of a lack of flexibility in the use of environmental cues? Hippocampus. 1999;9:668–681. [Abstract] [Google Scholar]
- Xiao C, Srinivasan R, Drenan RM, Mackey ED, McIntosh JM, Lester HA. Characterizing functional alpha6beta2 nicotinic acetylcholine receptors in vitro: mutant beta2 subunits improve membrane expression, and fluorescent proteins reveal responsive cells. Biochem Pharmacol. 2011;82:852–861. [Europe PMC free article] [Abstract] [Google Scholar]
- Xu Z, Seidler FJ, Cousins MM, Slikker W, Jr, Slotkin TA. Adolescent nicotine administration alters serotonin receptors and cell signaling mediated through adenylyl cyclase. Brain Res. 2002;951:280–292. [Abstract] [Google Scholar]
- Yamauchi J, Miyamoto Y, Murabe M, Fujiwara Y, Sanbe A, Fujita Y, Murase S, Tanoue A. Gadd45a, the gene induced by the mood stabilizer valproic acid, regulates neurite outgrowth through JNK and the substrate paxillin in N1E-115 neuroblastoma cells. Exp Cell Res. 2007;313:1886–1896. [Abstract] [Google Scholar]
- Yamazaki Y, Jia Y, Hamaue N, Sumikawa K. Nicotine-induced switch in the nicotinic cholinergic mechanisms of facilitation of long-term potentiation induction. Eur J Neurosci. 2005;22:845–860. [Abstract] [Google Scholar]
- Yeckel MF, Berger TW. Feedforward excitation of the hippocampus by afferents from the entorhinal cortex: redefinition of the role of the trisynaptic pathway. Proc Natl Acad Sci U S A. 1990;87:5832–5836. [Europe PMC free article] [Abstract] [Google Scholar]
- Yoshida K, Imura H. Nicotinic cholinergic receptors in brain synaptosomes. Brain Res. 1979;172:453–459. [Abstract] [Google Scholar]
- Yoshitake T, Yoshitake S, Savage S, Elvander-Tottie E, Ogren SO, Kehr J. Galanin differentially regulates acetylcholine release in ventral and dorsal hippocampus: a microdialysis study in awake rat. Neuroscience. 2011;197:172–180. [Abstract] [Google Scholar]
- Zahm DS. Functional-anatomical implications of the nucleus accumbens core and shell subterritories. Ann N Y Acad Sci. 1999;877:113–128. [Abstract] [Google Scholar]
- Zahm DS, Brog JS. On the significance of subterritories in the “accumbens” part of the rat ventral striatum. Neuroscience. 1992;50:751–767. [Abstract] [Google Scholar]
- Zarei MM, Radcliffe KA, Chen D, Patrick JW, Dani JA. Distributions of nicotinic acetylcholine receptor alpha7 and beta2 subunits on cultured hippocampal neurons. Neuroscience. 1999;88:755–764. [Abstract] [Google Scholar]
- Zhang TA, Tang J, Pidoplichko VI, Dani JA. Addictive nicotine alters local circuit inhibition during the induction of in vivo hippocampal synaptic potentiation. J Neurosci. 2010;30:6443–6453. [Europe PMC free article] [Abstract] [Google Scholar]
- Zhang ZW, Vijayaraghavan S, Berg DK. Neuronal acetylcholine receptors that bind alpha-bungarotoxin with high affinity function as ligand-gated ion channels. Neuron. 1994;12:167–177. [Abstract] [Google Scholar]
- Zhao M, Choi YS, Obrietan K, Dudek SM. Synaptic plasticity (and the lack thereof) in hippocampal CA2 neurons. J Neurosci. 2007;27:12025–12032. [Europe PMC free article] [Abstract] [Google Scholar]
- Zhou FM, Wilson CJ, Dani JA. Cholinergic interneuron characteristics and nicotinic properties in the striatum. J Neurobiol. 2002;53:590–605. [Abstract] [Google Scholar]
- Zhu XO, Brown MW, Aggleton JP. Neuronal signalling of information important to visual recognition memory in rat rhinal and neighbouring cortices. Eur J Neurosci. 1995;7:753–765. [Abstract] [Google Scholar]
- Zola-Morgan S, Squire LR, Amaral DG. Human amnesia and the medial temporal region: enduring memory impairment following a bilateral lesion limited to field CA1 of the hippocampus. J Neurosci. 1986;6:2950–2967. [Europe PMC free article] [Abstract] [Google Scholar]
- Zwart R, Vijverberg HP. Four pharmacologically distinct subtypes of alpha4beta2 nicotinic acetylcholine receptor expressed in Xenopus laevis oocytes. Mol Pharmacol. 1998;54:1124–1131. [Abstract] [Google Scholar]
Full text links
Read article at publisher's site: https://doi.org/10.1016/j.nlm.2013.08.004
Read article for free, from open access legal sources, via Unpaywall:
https://europepmc.org/articles/pmc3873373?pdf=render
Citations & impact
Impact metrics
Citations of article over time
Smart citations by scite.ai
Explore citation contexts and check if this article has been
supported or disputed.
https://scite.ai/reports/10.1016/j.nlm.2013.08.004
Article citations
Oral administration of coenzyme Q10 ameliorates memory impairment induced by nicotine-ethanol abstinence through restoration of biochemical changes in male rat hippocampal tissues.
Sci Rep, 14(1):11413, 18 May 2024
Cited by: 0 articles | PMID: 38762560 | PMCID: PMC11102461
Enhanced Novel Object Recognition and Spatial Memory in Rats Selectively Bred for High Nicotine Preference.
Brain Sci, 14(5):427, 25 Apr 2024
Cited by: 0 articles | PMID: 38790406 | PMCID: PMC11118842
Single nucleotide polymorphisms rs148582811 regulates its host gene ARVCF expression to affect nicotine-associated hippocampus-dependent memory.
iScience, 26(12):108335, 28 Oct 2023
Cited by: 1 article | PMID: 38025780 | PMCID: PMC10679859
ChAT::Cre transgenic rats show sex-dependent altered fear behaviors, ultrasonic vocalizations and cholinergic marker expression.
Genes Brain Behav, 22(1):e12837, 13 Jan 2023
Cited by: 1 article | PMID: 36636833 | PMCID: PMC9994175
Nomogram for Prediction of Postoperative Delirium after Deep Brain Stimulation of Subthalamic Nucleus in Parkinson's Disease under General Anesthesia.
Parkinsons Dis, 2022:6915627, 29 Nov 2022
Cited by: 2 articles | PMID: 36483978 | PMCID: PMC9726266
Go to all (37) article citations
Similar Articles
To arrive at the top five similar articles we use a word-weighted algorithm to compare words from the Title and Abstract of each citation.
Nicotinic modulation of hippocampal cell signaling and associated effects on learning and memory.
Physiol Behav, 155:162-171, 11 Dec 2015
Cited by: 30 articles | PMID: 26687895 | PMCID: PMC4718748
Review Free full text in Europe PMC
Nicotine and hippocampus-dependent learning: implications for addiction.
Mol Neurobiol, 34(2):93-107, 01 Oct 2006
Cited by: 50 articles | PMID: 17220532 | PMCID: PMC2716133
Review Free full text in Europe PMC
Effects of drugs of abuse on hippocampal plasticity and hippocampus-dependent learning and memory: contributions to development and maintenance of addiction.
Learn Mem, 23(10):515-533, 15 Sep 2016
Cited by: 122 articles | PMID: 27634143 | PMCID: PMC5026208
Review Free full text in Europe PMC
Modulation of hippocampus-dependent learning and synaptic plasticity by nicotine.
Mol Neurobiol, 38(1):101-121, 09 Aug 2008
Cited by: 161 articles | PMID: 18690555 | PMCID: PMC2683366
Review Free full text in Europe PMC
Funding
Funders who supported this work.
NCI NIH HHS (2)
Grant ID: P50 CA143187
Grant ID: CA143187
NIDA NIH HHS (5)
Grant ID: R01 DA017949
Grant ID: R01 DA024787
Grant ID: DA007237
Grant ID: DA024787
Grant ID: T32 DA007237
NIMH NIH HHS (1)
Grant ID: MH 073949