Abstract
Free full text

Global virus outbreaks: Interferons as 1st responders
Abstract
Outbreaks of severe virus infections with the potential to cause global pandemics are increasing. In many instances these outbreaks have been newly emerging (SARS coronavirus), re-emerging (Ebola virus, Zika virus) or zoonotic (avian influenza H5N1) virus infections. In the absence of a targeted vaccine or a pathogen-specific antiviral, broad-spectrum antivirals would function to limit virus spread. Given the direct antiviral effects of type I interferons (IFNs) in inhibiting the replication of both DNA and RNA viruses at different stages of their replicative cycles, and the effects of type I IFNs on activating immune cell populations to clear virus infections, IFNs-α/βpresent as ideal candidate broad-spectrum antivirals.
1. Introduction
Interferons (IFNs) are critical effectors of both innate and adaptive immune responses, associated with the development of immune cell populations and their activation to respond to pathogens, cancers and other insults. IFNs are classified according to the receptors through which they signal (Fig. 1 ). Type I IFNs (-α, -β, -δ, -ε, -ζ, -κ, -τ, and -ω), signal through the IFN-α/β receptor (IFNAR), and are one of three major classes of IFNs, the other two being type II IFN (-γ) and type III IFNs (-λ1, -λ2, -λ3). Type I IFNs were discovered for their effectiveness to inhibit virus replication [1], and have since been shown to exert critical effects on the development and activation of immune cell subsets. Given both their antiviral and immunomodulatory effects, type I IFNs, alone, or in combination with other therapies, have been examined clinically in a variety of chronic and acute viral infections. This review will highlight the antiviral and immunomodulatory effects of type I IFNs, the mechanisms by which viruses inhibit and evade a host type I IFN response, and describe recent therapeutic applications for recombinant type I IFNs in the treatment of viral infections.
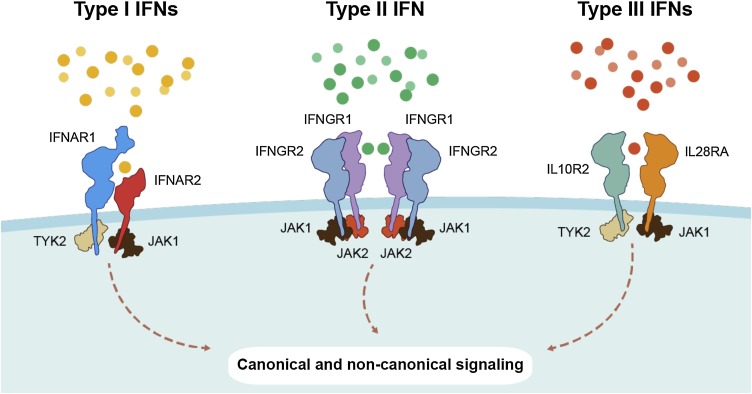
IFNs and their cognate receptors. IFNs are classified based on the receptors through which they signal. Type I IFNs (-α, -β, -δ, -ε, -ζ, -κ, -τ, and -ω) bind to and activate the IFN-α/β receptor (IFNAR) complex. Type II IFN (-γ) activates the IFN-γ receptor 1 and type III IFNs (-λ1, -λ2, -λ3) signal through a receptor complex made up of IL28RA and IL10R2.
1.1. Induction of type I IFNs, receptor activation and signaling
Type I IFNs are induced following detection of pathogen-associated molecular patterns (PAMPs) and damage/danger-associated molecular patterns (DAMPs) by innate pattern recognition receptors (PRRs). Expressed by both immune and non-immune cells, PRRs comprise Toll-like receptors (TLRs), C-type lectin receptors (CLRs), retinoic acid-inducible gene 1 (RIG-I)-like receptors (RLRs), nucleotide-binding oligomerization domain (NOD)-like receptors (NLRs), Aim2-like receptors (ALRs) and cyclic GMP-AMP synthase (cGAS), which together bind a diverse range of extracellular and endosomal PAMPs and DAMPs (Fig. 2 ). PRR signaling results in the expression of type I IFNs and pro-inflammatory cytokines mediated by three essential transcription factors: IFN regulatory factor (IRF)3, IRF7, and nuclear factor-κB (NF-κB) [[2], [3], [4], [5], [6]]. Virus-inducible IFN-β expression is upregulated by the formation of an IFN-β enhanceosome, which is comprised of NF-κB, IRF3, IRF7 and c-Jun at the IFN-β promoter [7,8]. The induction of type I IFNs provides the first line of defense against many diverse pathogens. Indeed, IFN dysregulation can lead to increased virus susceptibility: RIG-I-/- and MDA5-/- mice produce lower levels of type I IFNs and are more susceptible to infection by RNA viruses, including Japanese encephalitis virus (JEV), encephalomyocarditis virus (EMCV), and West Nile virus (WNV) [9,10].
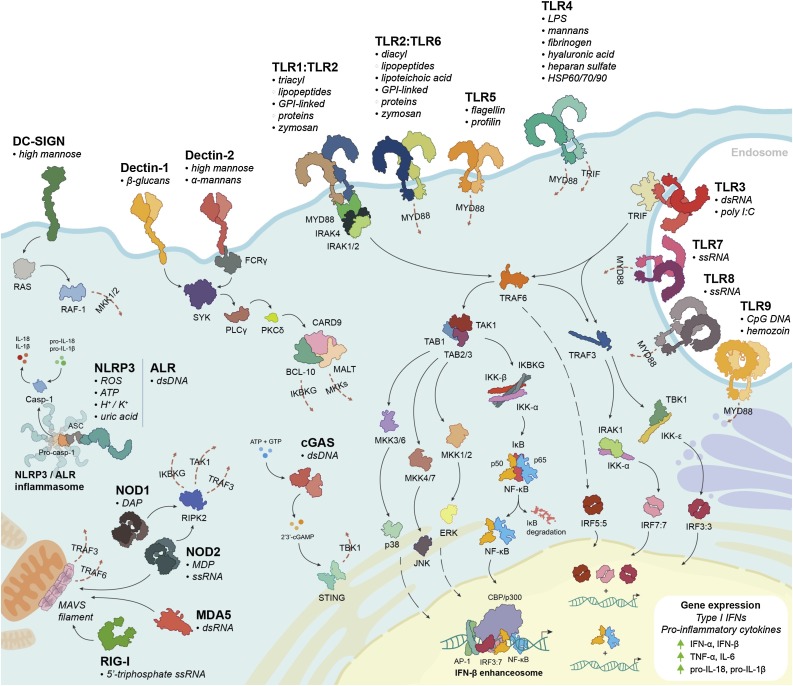
Pattern Recognition Receptor activation leads to type I IFN production. Binding of PAMPs and DAMPs to host PRRs (TLRs, CLRs, RLRs, NLRs, ALRs, and cGAS) induces signaling cascades that activate IRF3, IRF7 and NF-κB, resulting in the production of type I IFNs-α/β and pro-inflammatory cytokines.
Type I IFNs bind to their cognate transmembrane receptor, IFNAR, comprised of an IFN-α/β receptor alpha chain (IFNAR1) and an IFN-α/β receptor beta chain (IFNAR2). Receptor binding leads to activation of multiple intracellular signaling cascades (Fig. 3 ). Best known is activation of the canonical Janus kinase (JAK)-signal transducer and activator of transcription (STAT) pathway, whereby IFNAR-associated JAK1 and TYK2 participate in the recruitment of STATs (1–6) to IFNAR and their subsequent phosphorylation-activation to form homo- or heterodimers [[11], [12], [13], [14]]. Unlike other STAT dimers, STAT1-STAT2 heterodimers also bind IRF9 to form the IFN-stimulated gene (ISG) factor 3 (ISGF3) complex [15,16]. In the nucleus, ISGF3 binds to IFN-sensitive response elements (ISREs), 5′-AGTTTN3TTTC-3′ [15,16], while other STAT dimers bind to IFN-γ activated sequence (GAS) elements, 5′-TTCN3GAA-3′, to initiate transcription of ISGs [17]. Several non-canonical pathways are also activated by type I IFNs, including the p38-associated mitogen-activated protein kinase (MAPK) signaling pathway [18] to modulate histone modification and early gene expression [19], and the phosphoinositide 3-kinase (PI3K) and protein kinase B (AKT) pathway, to regulate mTORC1 activation, protein synthesis and cap-dependent mRNA translation [20][D. Saleiro et al, this issue].
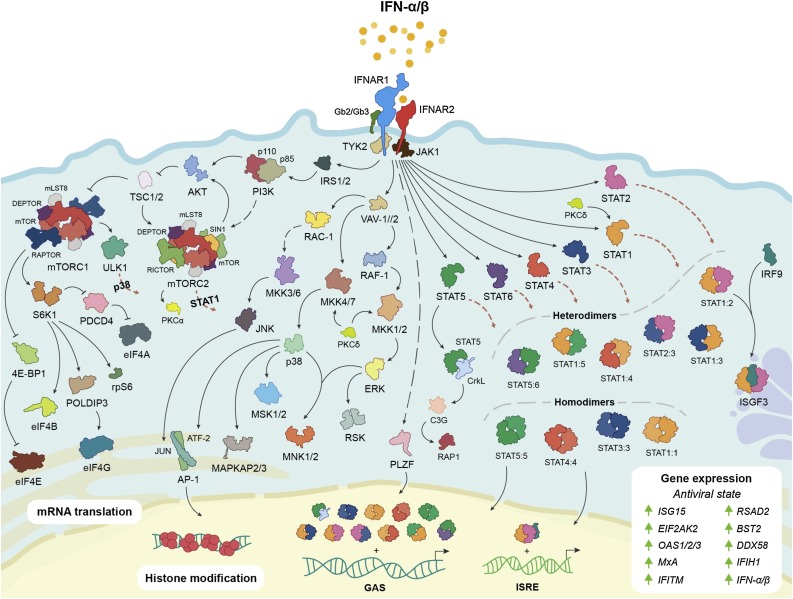
Type I IFN signaling. IFNs-α/β bind to IFNAR, inducing the phosphorylation-activation of tyrosine kinases JAK1 and TYK2. JAK1 and TYK2 activation initiates multiple canonical and non-canonical signaling cascades that are critical for the regulation of cellular processes and the expression of ISGs for the innate immune response.
In humans, IFN-inducible transcriptional and translational regulation of ISGs results in the expression of over 7000 genes, that contribute to cellular processes including metabolism, survival, migration, activation and, importantly, innate host defense against viral infections [21]. Notably, many ISGs have been identified with functions that interfere with different stages of viral replication and transmission (Table 1 ). Interestingly, in vitro studies that examined the effects of IFN-ß against Coxsackievirus B3 infection, identified a novel function of IFN-ß in regulating glucose metabolism, mediated by activation of the PI3K/AKT signaling pathway, important for the induction of a rapid antiviral response [59].
Table 1
Antiviral ISGs with known functions.
ISG(s) | Function(s) | Reference(s) |
---|---|---|
APOBEC3 | Cytidine deamination of single-stranded viral DNA (deoxycytidine to deoxyuridine) to inhibit retrovirus replication. | [22,23] |
BST2 | Binds and inhibits the release of budding progeny virions. | [24,25] |
DDX58 | RIG-I detects ssRNA to induce MAVS and IRF-dependent type I IFN production. | [9,[26], [27], [28]] |
DDX60 | Enhances RIG-I and MDA5-dependent type I IFN production. | [29,30] |
EIF2AK2 | Detects dsRNA and phosphorylates EIF2α to inhibit both cellular and viral mRNA translation. | [31,32] |
IFIH1 | Detects dsRNA to induce MAVS and IRF-dependent type I IFN production. | [26,28] |
IFITM1, IFITM2, and IFITM3 | Inhibit viral entry. IFITM3 inhibits the formation of fusion pores in the late endosome. | [[33], [34], [35]] |
IRF1 and IRF7 | Induce ISG expression in the absence of type I IFN signaling. | [36,37] |
ISG15 | Regulates host and viral protein function by ISGylation. | [[38], [39], [40]] |
ISG20 | Cleaves ssRNA to inhibit viral RNA synthesis and replication. | [41,42] |
MX1 | Forms oligomeric ring structures that bind viral nucleoproteins to inhibit replication. | [[43], [44], [45]] |
OAS1, OAS2, and OAS3 | Detect dsRNA and synthesize 2′–5′ olygoadenylates, which are the substrate for RNaseL activation. | [[46], [47], [48]] |
OASL | Enhances RIG-I activation. | [49] |
RSAD2 | Restricts viral budding by modulating lipid synthesis. | [50] |
SAMHD1 | Depletes intracellular dNTPs to inhibit viral replication. | [[51], [52], [53], [54]] |
TRIM5 | Binds virus capsid proteins to inhibit viral infection. | [55] |
TRIM25 | Ubiquitinates RIG-I to enhance type I IFN induction. | [56] |
ZC3HAV1 | Inhibits viral mRNA expression and enhances RIG-I-dependent type I IFN induction. | [57,58] |
1.2. Effects of type I IFNs on the immune system
Type I IFNs have diverse effects on the immune system, beyond the induction of antiviral ISGs, ranging from regulation of leukocyte development and differentiation, to immune cell recruitment and activation. At the earliest stages of leukocyte development, IFN-α signaling has been shown to affect the renewal and proliferation of hematopoietic stem cells (HSCs) [60,61]. In mice, IFN-α enhances the proliferation of dormant HSCs in a STAT1- and AKT-phosphorylation dependent manner [60]. Moreover, chronic IFN-α signaling can lead to HSC exhaustion, resulting in a reduction in the number of quiescent HSCs in the bone marrow [60]. As a result, HSCs that lack the negative regulator of type I IFN signaling, IRF2, fail to outcompete IRF2+/- HSCs in competitive repopulation assays [61].
Type I IFNs also regulate the expression of chemokines and cell adhesion receptors, thereby affecting the trafficking of different immune cell populations. IFN-α/β signaling upregulates chemokine (C-C motif) ligand (CCL) 2 [62], CCL3, CCL4 [63], CCL5 [64], CCL7 [65], CCL12 [66], chemokine (C-X-C motif) ligand (CXCL) 9 [67], CXCL10 [66,67], CXCL11 [68] and cluster of differentiation (CD) 69 [69], while downregulating the expression of CXCL1, CXCL2 [[60], [61], [62], [63], [64], [65], [66], [67], [68], [69], [70], [71], [72]]. Briefly, CCL2, CCL7 and CCL12 are chemoattractants for monocytes [62,73], while CCL5, CXCL9, CXCL10, and CXCL11 are chemoattractants for T cells [74,75] – CCL2 also recruits memory T cells [76]. CCL3 and CCL4 are chemoattractants for monocytes and macrophages [77], and CXCL1 and CXCL2 recruit neutrophils [70]. IFN-α/β-inducible CD69 expression promotes the retention of lymphocytes in lymph nodes by inhibiting sphingosine 1-phosphate receptor-1 (S1P1) [69], thereby promoting antigen presentation and lymphocyte activation.
In addition to influencing chemokine expression, type I IFNs also regulate the survival and activation of innate and adaptive immune cells. Although type I IFNs inhibit the recruitment of neutrophils by suppressing CXCL1 and CXCL2 expression, IFN-α has been shown to promote neutrophil survival by inducing the expression of cellular inhibitor of apoptosis 2 (cIAP2) via STAT1 and STAT3 [78]. In NK cells, type I IFN signaling enhances IFN-γ production [79], cell survival [80], and cytotoxicity against tumor cells and virus-infected cells [[80], [81], [82], [83]] through upregulation of Fas ligand (FasL) expression [84]. Moreover, type I IFN signaling in macrophages is important for phagocytosis [85] and nitric oxide synthase 2 (NOS2) expression [66], both of which contribute to the clearance of pathogens, tumor cells and damaged tissues.
Monocytes express high levels of IFNAR on their cell surface [86], and in the presence of IFN-α and granulocyte-macrophage colony-stimulating factor (GM-CSF), rapidly differentiate into DCs that are capable of presenting antigens, priming CD4+ T helper 1 (Th1) cells, and activating CD8+ T cells [[87], [88], [89], [90]]. Type I IFN signaling in conventional DCs (cDCs) further directs Th1 immunity and T cell activation by boosting IL-12 production in the presence of PAMPs [91]. IFN-α/β enhances the expression of major histocompatibility complex (MHC) class I, MHC class II, and the co-stimulatory factors: CD40, CD80, and CD86 [92,93]. Plasmacytoid DCs (pDCs) also produce considerably more IFN-α/β in the presence of PAMPs when compared to other leukocytes, due to high levels of constitutive IRF7 expression [94,95].
Type I IFNs regulate effector and memory CD4+ and CD8+ T cells [reviewed in [96]]. For cytotoxic CD8+ T cells, IFNs-α/β upregulate IFN-γ, perforin and granzyme B expression [97]. Curtsinger et al. [97] showed that in the absence of IFN-α and IL-12 signaling, CD8+ T cells fail to upregulate perforin or granzyme B expression even in the presence of antigen and co-stimulation; therefore, IFN-α and IL-12 may provide a necessary third signal for CD8+ T cell effector function. In the context of viral infections, type I IFNs are also critical for CD8+ T cell clonal expansion and memory formation [[98], [99], [100]], and contribute to Eomesodermin (Eomes) and T-box transcription factor TBX21 (T-bet) expression [101]. Using IFNAR -/- cells, Le Bon et al. [102] showed that intact type I IFN signaling affects the ability for CD4+ T cells to provide B cell help, as well as the formation of antigen-specific antibody responses. In regard to other T cell subsets, type I IFNs downregulate both Th2 and Th17 differentiation by inhibiting GATA3 and IL-17 expression, respectively [103,104].
IFN-β-/- mice exhibit a defect in B cell maturation resulting in significantly fewer circulating immunoglobulin (Ig) M+ B cells than in wild-type mice [105]. This defect is characterized by a reduction in pro-B cell and B220+, IgM+ and CD23+ B cell populations in the bone marrow. Moreover, IFN-β-/- B220+ B cells express significantly lower levels of IgM and CD23 than wild-type B220+ B cells [105]. Like monocytes, B cells also express high levels of surface IFNAR [86], and IFN-α/β signaling is important for B cell survival and activation. Type I IFN signaling upregulates the surface expression of MHC class I, MHC class II, L-selectin, CD69, CD86, and CD25 on B cells [86,106,107], which prime them for B cell receptor (BCR) activation [105]. IFN-β expression by transitional stage 1 (T1) B cells in the spleen is critical for their survival and development [108]. Furthermore, IFN-α induces B cell activating factor (BAFF) and a proliferation-inducing ligand (APRIL) expression by DCs [[109], [110], [111]], which promote B cell Ig class switching [112,113]. Virus-activated pDCs also direct antigen-specific B cell differentiation into Ig-secreting plasma cells mediated by a combination of type I IFN and IL-6 signaling [114].
2. Viral antagonism of type I IFN responses
Given the effectiveness of IFN-inducible antiviral responses in limiting infection and viral replication in a largely cell-independent manner, irrespective of the virus, viruses have developed different mechanisms to inhibit type I IFN induction and signaling, and the activity of antiviral ISGs (Table 2 ). Viruses may encode in their genomes multiple different proteins that target different facets of type I IFN-inducible antiviral responses, or a single multifunctional protein: herpes simplex virus (HSV) encodes several proteins - ICP0, ICP27, ICP34.5, US11 - directed against different IFN-inducible targets, whereas influenza A virus (IAV), encodes a multifunctional viral protein - NS1 - with the capacity to interfere with multiple host pathways and proteins involved in an antiviral response.
Table 2
Virus-encoded proteins that antagonize the type I IFN response.
Virus | Viral Protein(s) | Function(s) | Reference(s) |
---|---|---|---|
Chikungunya virus (CHIKV) | nsP2 | Inhibits type I IFN-inducible JAK-STAT signaling. | [115] |
Coxsackievirus | 2A protease | Cleaves MAVS and MDA5 to block type I IFN induction. | [116] |
3C protease | Cleaves RIG-I, MAVS and TRIF to block type I IFN induction. | [116,117] | |
Dengue virus (DENV) | NS2A, NS4A and NS4B | Inhibit IFN-β-inducible STAT1 phosphorylation and ISG expression. | [118,119] |
NS2B and NS3 | Inhibit type I IFN production. | [120] | |
NS5 | Binds STAT2 and inhibits IFN-α-inducible STAT2 phosphorylation. | [121] | |
Ebola virus (EBOV) | VP24 | Binds STAT1 and karyopherin-α1 to inhibit nuclear translocation of phosphorylated STAT1. | [122,123] |
VP35 | Binds dsRNA to suppress RLR-dependent IRF3 activation and IFN-β induction. | [124,125] | |
Epstein-Barr virus (EBV) | BRLF1 | Inhibits IRF3 and IRF7 to suppress IFN-β induction. | [126] |
BZLF1 | Inhibits IRF7 activation to suppress IFN-β induction. | [127] | |
LF2 | Binds IRF7 to inhibit IFN-α induction. | [128] | |
Hepatitis B virus (HBV) | HBx | Binds RIG-I, TRAF3 and MAVS to inhibit type I IFN induction. | [129] |
Pol | Binds karyopherin-α and PKC-δ to inhibit IFN-α-inducible STAT1 phosphorylation and nuclear translocation of STAT1-STAT2 heterodimers. Also binds DDX3 to inhibit TBK1 and IKKε-dependent type I IFN induction. | [130,131] | |
Hepatitis C virus (HCV) | Core protein | Induces SOCS3 expression to inhibit IFN-α-inducible STAT1 phosphorylation. | [132] |
E2 | Inhibits PKR activation. | [133] | |
NS3 and NS4A | Inhibit TLR3 and MAVS-dependent IRF3 activation and IFN-β induction. | [134,135] | |
NS5A | Binds PKR to inhibit PKR dimerization. Also binds STAT1 to inhibit IFN-α-inducible STAT1 phosphorylation and ISG expression. | [136,137] | |
Human cytomegalovirus (HCMV) | IE72 | Interacts with STAT2 to inhibit ISGF3 binding to ISREs. | [138,139] |
IE86 | Inhibits IFN-β production. | [140] | |
pIRS1 and pTRS1 | Bind dsRNA to inhibit PKR and OAS activation. | [141] | |
pUL26 | Inhibits ISGylation. | [142] | |
Human immuno-deficiency virus (HIV) | Tat | Inhibits PKR activation. | [143] |
Vif | Inhibits APOBEC3G mRNA translation and enhances its post-translational degradation. | [144] | |
Vpu | Inhibits the antiviral activity of tetherin. | [24] | |
Vpx | Enhances degradation of SAMHD1. | [51] | |
Human parainfluenza virus (HPIV) | C | Inhibits type I IFN production and signaling. | [145] |
V | Inhibits type I IFN production and contributes to STAT2 degradation. | [146] | |
Human papillomavirus (HPV) | E6 and E7 | Inhibit ISG expression. E6 inhibits type I IFN-inducible STAT1 phosphorylation and ISRE activation. Also, E6 binds IRF3 to inhibit type I IFN production. | [147,148] |
Human respiratory syncytial virus (HRSV) | NS1 and NS2 | Inhibit the activation and nuclear translocation of IRF3 to inhibit type I IFN induction. | [149] |
Human rhinovirus (HRV) | - unknown - | HRV induces minimal IRF3 activation and IFN-β production in untreated cells in comparison to cells treated with cycloheximide. | [150] |
Herpes simplex virus (HSV) | ICP0 | Inhibits IRF3 activation. | [151] |
ICP27 | Inhibits IFN-α-inducible STAT1 phosphorylation and nuclear translocation. | [152] | |
ICP34.5 | Reverses PKR-dependent eIF2α phosphorylation. | [153] | |
US11 | Binds dsRNA to inhibit PKR and RNaseL activation. | [154] | |
Influenza A virus (IAV) | NS1 | Binds RIG-I, CPSF4, and PABPII to suppress type I IFN production. Binds dsRNA to inhibit PKR and RNaseL activation. Inhibits IFNAR1 expression and IFN-β-inducible STAT phosphorylation. Induces SOCS1 expression. | [[155], [156], [157], [158], [159], [160]] |
Influenza B virus (IBV) | NS1 | Inhibits type I IFN production and binds dsRNA to inhibit PKR activation. | [161] |
Japanese encephalitis virus (JEV) | NS4A | Inhibits type I IFN-inducible STAT1 and STAT2 phosphorylation, and ISRE activation. | [162] |
NS5 | Inhibits type I IFN-inducible STAT1 phosphorylation and ISG expression. | [163,164] | |
Lassa virus (LASV) | NP | Inhibits the nuclear translocation of IRF3 and IFN-β induction. | [165,166] |
Lymphocytic choriomeningitis virus (LCMV) | NP | Binds RIG-I and MDA5. Inhibits the nuclear translocation of IRF3 and IFN-β induction. | [166,167] |
Marburg virus (MARV) | VP24 | Inhibits type I IFN-inducible STAT1 and STAT2 phosphorylation. | [168] |
VP35 | Binds dsRNA to suppress RLR-dependent IRF3 activation and IFN-β induction. | [169] | |
VP40 | Inhibits type I IFN-inducible STAT1 phosphorylation. | [170] | |
Measles virus (MeV) | C and P | Inhibit IFN-α-inducible ISRE activation. | [171] |
N | Inhibits nuclear translocation of STAT1 and STAT2. | [171] | |
V | Binds STAT2 to inhibit type I IFN-inducible ISRE induction. | [172] | |
Middle East respiratory syndrome coronavirus (MERS-CoV) | M, ORF4b and ORF5 | Inhibit IRF3 activation and IFN-β induction. Also inhibit ISRE activation. | [173] |
ORF4a | Binds dsRNA to inhibit RIG-I and MDA5-dependent IFN-β induction. Also inhibits ISRE activation. | [173,174] | |
Mumps virus (MuV) | V | Inhibits IFN-β-inducible STAT1 and STAT2 phosphorylation. | [175] |
Nipah virus (NiV) | V, P and W | Inhibit IFN-β-inducible STAT1 phosphorylation and ISRE activation. | [176] |
Poliovirus (PV) | 2A protease | Cleaves MAVS and MDA5 to block type I IFN induction. | [116] |
3C protease | Cleaves RIG-I to block type I IFN induction. | [116] | |
Rabies virus (RABV) | P | Binds STAT1, STAT2, and STAT3 to inhibit nuclear translocation of phosphorylated STAT proteins, and ISRE and GAS activation. Also inhibits IRF3 activation and IFN-β induction. | [[177], [178], [179]] |
Rotavirus (RV) | NSP1 | Enhances IRF3 and IRF7 degradation to inhibit type I IFN induction. Also inhibits NF-B activation. | [180,181] |
Severe acute respiratory syndrome coronavirus (SARS-CoV) | M | Binds RIG-I, TRAF3, TBK1 and IKKε to inhibit IRF3 and IRF7-dependent ISRE activation, and type I IFN production. | [182,183] |
Nsp1 | Enhances host mRNA degradation and inhibits mRNA translation to suppress type I IFN expression. Also inhibits IRF3 and IRF7 activation, and IFN-α-inducible STAT1 phosphorylation. | [[184], [185], [186]] | |
Nsp3 | Inhibits IRF3 phosphorylation and nuclear translocation. | [187] | |
ORF6 | Binds karyopherin-α2 and -β1 to inhibit nuclear translocation of STAT1, and ISG expression. | [188] | |
Vaccinia virus (VACV) | E3L | Binds dsRNA to inhibit PKR and RNaseL activation. Also inhibits IRF3 activation and IFN-β induction. | [189] |
K3L | Inhibits PKR activation. | [190] | |
vIFN-α/βRc | Binds type I IFN to inhibit IFN signaling. | [191] | |
West Nile virus (WNV) | NS4B | Inhibits type I IFN-inducible STAT1 phosphorylation and ISRE activation. | [119] |
NS5 | Inhibits IFN-β-inducible STAT1 phosphorylation and ISG expression. | [192,193] | |
Yellow fever virus (YFV) | NS4B | Inhibits type I IFN-inducible STAT1 phosphorylation and ISRE activation. | [119] |
NS5 | Binds STAT2 to inhibit ISGF3 binding to ISREs. | [194] | |
Zika Virus (ZIKV) | NS1, NS4A, NS5 | Inhibit type I IFN induction and signaling. | [195] |
3. Type I IFNs as broad-spectrum antivirals
Newly emerging and re-emerging virus infections pose a serious threat to global health (Fig. 4 ). In the absence of targeted vaccines for newly emerging virus infections, or sufficient vaccine availability for re-emerging virus infections, there is an obvious need for direct antivirals to deploy during a pandemic. Viruses mutate to become resistant to pathogen-specifc antivirals, necessitating the development of broad-spectrum pleiotropic antivirals. Type I IFNs are prototypical candidate broad-spectrum antivirals, specifically because of their rapid induction in response to any and all virus infections, the pleiotropic nature of their effects on inhibition of different stages of viral replicative cycles and their effects on activating immune cells to clear virus infections. Not surprisingly, therefore, as mentioned above, viruses have evolved to evade an IFN response, specifically because it is so critical for the host immune response to infection. However, given the pleiotropic nature of the antiviral effects of IFNs, virus-targeted inhibition of some of these elements may still permit a partial - and effective - IFN response. To date, however, type I IFNs have seen limited clinical use for the treatment of acute and chronic infections.
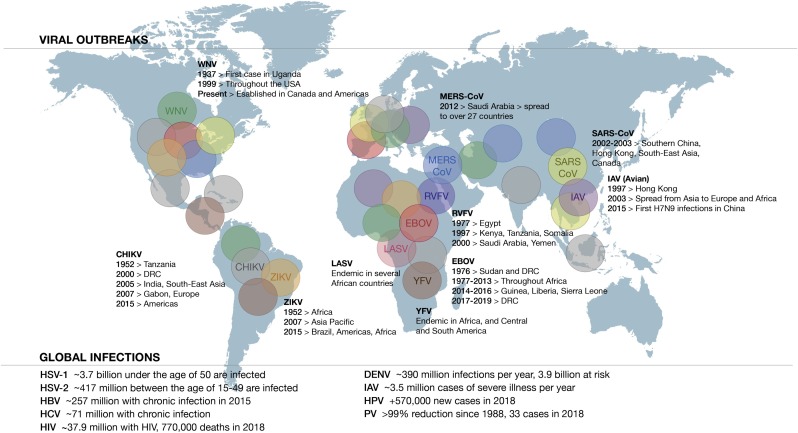
Extent of global viral infections. Graphical depiction of global viral outbreaks and summary of the impact of global viral infections based on information gathered from the World Health Organization (WHO) [196]. CHIKV (grey), chikungunya virus; EBOV (red), Ebola virus; IAV (purple), influenza A virus; MERS-CoV (blue), Middle-East respiratory syndrome coronavirus; LASV (pink), Lassa virus; RVFV (blue-purple), Rift Valley fever virus; SARS-CoV (yellow-green), severe acute respiratory syndrome coronavirus; WNV (green), West Nile virus; YFV (brown), yellow fever virus; ZIKV (gold), Zika virus; DENV, dengue virus; HBV, hepatitis B virus; HCV, hepatitis C virus; HIV, human immunodeficiency virus; HPV, human papillomavirus; HSV-1/2, herpes simplex virus type 1/2; PV, poliovirus.
Pegylated recombinant IFN-α-2a/2b (PEG-INF) in combination with ribavirin, a nucleoside inhibitor of viral RNA synthesis, remains the standard of care for treatment for chronic hepatitis C virus (HCV) infection in those jurisdictions where direct antiviral agents (DAA) are unavailable. Type I IFNs were first approved for single-agent therapeutic use in the context of HCV infection in the 1990s. A major limitation for the clinical use of type I IFNs for HCV has been the prevalence of adverse events, with the most common adverse events including fatigue, headache, pyrexia, rigors, myalgia, nausea, abdominal pain, anxiety, depression, psychosis and insomnia [197]. Serious adverse events include neutropenia, thrombocytopenia, hyper- and hypothyroidism, pancreatitis, type I diabetes mellitus, and irreversible pulmonary hypertension [[198], [199], [200]]. Notably, these adverse advents are associated with sustained IFN treatment. Pegylation of recombinant IFN improved pharmacokinetics to allow for longer dosing intervals [201]. The clinical efficacy of IFN therapy is determined by measuring serum HCV RNA levels, where a sustained virological response (SVR) is defined by undetectable HCV RNA levels at 24 weeks following the completion of the IFN therapy. Patients infected with HCV genotype (G)2, G2, or G3, exhibit SVR rates of between 76–82% [[202], [203], [204], [205]], in comparison to SVR rates of approximately 67% and 40–50% for patients infected with HCV G4 and G1, respectively [[206], [207], [208], [209], [210], [211]]. Furthermore, patients with HCV G1/G2/G3 infections who exhibit a rapid virological response (RVR), defined by undetectable serum HCV RNA after 4 weeks of treatment, are most likely to achieve a SVR [212,213]. In addition to RVR, other predicators of SVR include low expression of the suppressor of cytokine signaling, SOCS3, mRNA and protein in the liver [214], whereas elevated SOCS3 expression [215], and polymorphisms in MX1 and IFNAR1 gene promoter regions are associated with non-response [216,217]. A lack of variation in the gene sequence encoding the PKR-binding domain within E2 and NS5A of HCV G1/G4 may contribute to the increased resistance to PEG-INF and ribavirin combination therapy [218].
PEG-INF has also shown therapeutic efficacy in patients with chronic hepatitis B virus (HBV) infection, as evidenced by seroconversion from hepatitis B e-antigen (HBeAg) positivity (active HBV replication) to HBeAg negativity and detectable levels of anti-HBe antibodies [[219], [220], [221]]. In a study conducted by Keating et al. [221], 42% of HBV infected patients treated with PEG-INF achieved seroconversion, while 12% cleared the virus one year following onset of treatment. In the same study, inactive HBV was detected in another 17% of treated patients.
In a single patient case study of chronic hepatitis E virus (HEV) infection, weekly PEG-INF treatment resulted in a decrease in serum HEV RNA by week 2 and a complete virological response by week 4 [222]. In the same patient, serum HEV RNA remained undetectable after 5 months. An SVR was seen in another patient with chronic HEV infection following PEG-INF treatment, with undetectable serum HEV RNA by week 3 that persisted for 6 months after cessation of PEG-INF treatment [223]. PEG-INF treatment induces ISG transcription and clearance of HEV infection in humanized mice [224].
Apart from their clinical use for chronic virus infections, little consideration has been given to the application of type I IFNs for severe acute virus infections. Between November 2002 and August 2003 over 8000 cases of severe acute respiratory syndrome coronoavirus (SARS-CoV) infection were reported worldwide, resulting in 916 deaths [196]. In the absence of any vaccine or approved DAAs, treatment was limited to corticosteroids, ribavirin and supportive care [225]. IFN alfacon-1 is a synthetic IFN-α, engineered such that at each position along the 165 amino acids of the protein the most frequently represented amino acid among all the IFN-α subtypes is present [226]. Results from in vitro and in vivo studies identify that IFN alfacon-1 consistently exhibits superior antiviral potency compared with other IFN-α subtypes, attributed to its higher binding affinity for IFNAR [[226], [227], [228]]. During the SARS outbreak of 2003, in a pilot clinical study in Toronto, Canada, evidence was provided that treatment with IFN alfacon-1 was associated with more rapid resolution of radiographic lung abnormalities and better oxygen saturation compared with those infected patients who received only corticosteroids [229]. IFN-treated patients exhibited lower levels of creatine kinase and more rapid return of lactate dehyrogenase levels to normal, indicative of improvement to SARS-associated lung parenchymal disease. Notably, given the short treatment time of 10 days, patients tolerated IFN treatment well with minimal adverse events and no exacerbation of any virus-associated symptoms. The single clinical adverse event reported was fever, likely associated with the underlying disease. Subsequent in vitro studies using human bronchial epithelial cells revealed that type I IFN treatment directly inhibits SARS-CoV replication [230,231], overriding the inhibitory effects of the virally encoded factors Nsp1, Nsp3 and ORF6. In non-human primate studies, administration of IFN prior to SARS-CoV infection in cynomolgus macaques limited viral replication and pulmonary damage [226].
Most recently, during the unprecedented 2013–2016 Ebola virus (EBOV) disease outbreak in West Africa, there were no approved therapies or vaccine available. Earlier studies in non-human primates provided evidence that IFN-α/β treatment reduced blood viremia and prolonged the survival of infected animals, [232,233], this despite the viral encoded IFN inhibitory factors, VP24 and VP35. Moreover, when combined with a monoclonal antibody cocktail directed against EBOV glycoproteins (ZMab), adenovirus-vectored IFN-α therapy extended the treatment window post-infection of non-human primates and suppressed viremia, leading to survival and robust immune responses [234]. Using transcription-competent virus-like particles (trVLP) to model EBOV Zaire infection in vitro, requiring only level 2 containment, McCarthy et al. screened a panel of candidate antiviral drugs and assessed their ability to suppress viral replication in human 293T cells [235]. IFN-α and IFN-β, along with the estrogen receptor modulator, toremifene, and number of viral polymerase inhibitors - lamivudine, zidovudine, tenofovir, and favipiravir - inhibited trVLP replication when administered 24
h post-infection. Notably, IFN-ß exhibited the most potent antiviral activity. Two- and three-drug combinations were tested using the same in vitro model. The two- and three-drug combinations that most potently inhibited EBOV trVLP replication were IFN-β + lamivudine, and IFN-β + lamivudine
+
zidovudine, respectively. Moreover, these same drug combinations inhibited infectious recombinant EBOV Zaire replication in 293
T cells [235]. Given the superior antiviral potency of IFN-β in both the trVLP studies and when used against EBOV Zaire, IFN-β was administered to 9 patients infected with EBOV in a proof-of-concept, single arm clinical study in Guinea, during the EBOV disease outbreak [236]. When compared to EBOV infected patients who only received standard supportive care at the same treatment facility, IFN-β treatment was associated with faster viral clearance from the blood, earlier resolution of disease symptoms and better survival [235]. Patients tolerated IFN treatment well with no exacerbated disease symptoms. These preliminary clinical findings provide a rationale for further clinical evaluation of IFN-ß for the treatment of EBOV infection.
Type I IFNs have also been shown to inhibit acute influenza A virus (IAV) infections, in vitro [155,237]. In primary human lung explants, IFN alfacon-1 inhibited avian H5N1 and pandemic H1N1 IAV replication, while upregulating the expression of several antiviral ISGs - PKR, OAS, and ISG15 [155]. Treatment of IAV-infected human lung A549 cells with IFN-β inhibits viral replication in a dose-dependent manner [237]. In the context of another acute virus infection, West Nile Virus, Type I IFNs have been shown to increase incorporation of microRNAs (miRNAs) into extracellular vesicles in A549 cells [238]. These enriched miRNAs regulate genes involved in antiviral and pro-inflammatory responses.
The orally administered, low molecular weight IFNAR2 agonist CDM-3008, has been shown to mimic the action of IFN-α by inhibiting both HCV [239] and HBV [240] infection in vitro. CDM-3008 is able to induce JAK-STAT signaling and upregulate ISG expression. Adenovirus-vectored IFN-alfacon-1, which has long-lasting antiviral activity, protects mice, hamsters and non-human primates in animal models of enterovirus (EV) 71 [241], EBOV [234,242], Chikungunya virus (CHIKV) [243], and Rift Valley fever virus (RVFV) infection [244]. A single dose of adenovirus-vectored IFN-α (DEF201), given intranasally within 6h post-RVFV challenge, significantly reduced viral loads in the serum, liver and spleen of hamsters [244]. DEF201, when administered prophylactically 21 days to 24
h prior to CHIKV challenge in mice, reduced CHIKV viral titers [243]. Furthermore, administration of DEF201 at 6
h and 12
h post-lethal EV71 infection of mice resulted in full and partial protection, respectively [241]. Viewed together, these in vivo studies provide a rationale for the evaluation of the prophylactic and therapeutic effects of adenovirus-vectored IFN-α for the treatment of severe acute virus infections when vaccines and/or approved antiviral agents are unavailable.
4. Concluding remarks
The preceding serves to illustrate the pleiotropic nature of type I IFNs in inhibiting virus replication, irrespective of the virus. Virus outbreaks pose a serious threat to global health, as exemplified by the recent outbreaks of SARS CoV, avian H5N1 influenza, Zika virus, WNV and EBOV. In the absence of a vaccine targeted against a newly emerging or re-emerging virus, antiviral drugs serve to limit viral spread. Viruses mutate to specifically evade pathogen-specific antivirals, a case in point being the emergence of Tamiflu-resistant influenza N1 strains [245]. A preferred strategy to limit virus outbreaks would be to deploy broad-spectrum antiviral agents that would exhibit pleiotropic effects [21], including invoking metabolic events important for the induction of a rapid antiviral response [59], targeting different stages of a virus replicative cycle and also invoking a robust immune response against the virus, regardless of the virus. Type I IFNs present as ideal broad-spectrum antiviral candidates and in limited clinical studies have demonstrated therapeutic effectiveness against severe acute virus infections. Their further evaluation is warranted.
References
Full text links
Read article at publisher's site: https://doi.org/10.1016/j.smim.2019.101300
Read article for free, from open access legal sources, via Unpaywall:
https://europepmc.org/articles/pmc7128104?pdf=render
Citations & impact
Impact metrics
Article citations
The Topical Novel Formulations of Interferon α-2в Effectively Inhibit HSV-1 Keratitis in the Rabbit Eye Model and HSV-2 Genital Herpes in Mice.
Viruses, 16(6):989, 19 Jun 2024
Cited by: 0 articles | PMID: 38932280 | PMCID: PMC11209562
Vascular dysfunction in hemorrhagic viral fevers: opportunities for organotypic modeling.
Biofabrication, 16(3), 05 Jun 2024
Cited by: 0 articles | PMID: 38749416 | PMCID: PMC11151171
Review Free full text in Europe PMC
Interferon β-1a ring prophylaxis to reduce household transmission of SARS-CoV-2: a cluster randomised clinical trial.
EClinicalMedicine, 62:102082, 20 Jul 2023
Cited by: 2 articles | PMID: 37538539
The Cytomegalovirus M35 Protein Directly Binds to the Interferon-β Enhancer and Modulates Transcription of Ifnb1 and Other IRF3-Driven Genes.
J Virol, 97(6):e0040023, 08 Jun 2023
Cited by: 2 articles | PMID: 37289084 | PMCID: PMC10308904
Reconsideration of interferon treatment for viral diseases: Lessons from SARS, MERS, and COVID-19.
Int Immunopharmacol, 121:110485, 16 Jun 2023
Cited by: 0 articles | PMID: 37348227 | PMCID: PMC10272952
Review Free full text in Europe PMC
Go to all (73) article citations
Similar Articles
To arrive at the top five similar articles we use a word-weighted algorithm to compare words from the Title and Abstract of each citation.
Antiviral Activity of Type I, II, and III Interferons Counterbalances ACE2 Inducibility and Restricts SARS-CoV-2.
mBio, 11(5):e01928-20, 10 Sep 2020
Cited by: 113 articles | PMID: 32913009 | PMCID: PMC7484541
Pathogenic influenza viruses and coronaviruses utilize similar and contrasting approaches to control interferon-stimulated gene responses.
mBio, 5(3):e01174-14, 20 May 2014
Cited by: 192 articles | PMID: 24846384 | PMCID: PMC4030454
Virucidal activity of a scorpion venom peptide variant mucroporin-M1 against measles, SARS-CoV and influenza H5N1 viruses.
Peptides, 32(7):1518-1525, 19 May 2011
Cited by: 74 articles | PMID: 21620914 | PMCID: PMC7115635
The yin and yang of viruses and interferons.
Trends Immunol, 33(4):190-197, 07 Feb 2012
Cited by: 80 articles | PMID: 22321608 | PMCID: PMC7106503
Review Free full text in Europe PMC