Abstract
Free full text

Evolutionary Insights into the Microneme-Secreted, Chitinase-Containing High-Molecular-Weight Protein Complexes Involved in Plasmodium Invasion of the Mosquito Midgut
ABSTRACT
While general mechanisms by which Plasmodium ookinetes invade the mosquito midgut have been studied, details regarding the interface of the ookinete, specifically its barriers to invasion, such as the proteolytic milieu, the chitin-containing, protein cross-linked peritrophic matrix, and the midgut epithelium, remain to be understood. Here, we review our knowledge of Plasmodium chitinases and the mechanisms by which they mediate ookinetes crossing the peritrophic matrix. The integration of new genomic insights into previous findings advances our understanding of Plasmodium evolution. Recently obtained Plasmodium species genomic data enable identification of the conserved residues in the experimentally demonstrated hetero-multimeric, high-molecular-weight complex comprised of a short chitinase covalently linked to binding partners, von Willebrand factor A domain-related protein (WARP) and secreted ookinete adhesive protein (SOAP). Artificial intelligence-based high-resolution structural modeling using the DeepMind AlphaFold algorithm yielded highly informative three-dimensional structures and insights into how short chitinases, WARP, and SOAP may interact at the atomic level to form the ookinete-secreted peritrophic matrix invasion complex. Elucidating the significance of the divergence of ookinete-secreted micronemal proteins among Plasmodium species may lead to a better understanding of the ookinete invasion machinery and the coevolution of Plasmodium-mosquito interactions.
INTRODUCTION
INTERACTIONS OF PLASMODIUM WITH THE MOSQUITO MIDGUT HAVE CHANGED OVER EVOLUTIONARY HISTORY
Mosquitoes are the definitive host of Plasmodium species, given that sexual reproduction takes place in the mosquito midgut (1). Plasmodium spp. that infect mammals and birds complete their life cycle in dipteran vectors, Anopheles spp. for mammal-infecting Plasmodium spp. and Anopheles spp. and/or culicine mosquitoes for avian-infecting Plasmodium species (1, 2). The long evolutionary history of the Plasmodium genus has led to biological differences in parasite-vector relationships, even among those species that infect the more closely related humans and nonhuman primates as opposed to rodents, birds, and reptiles (3). There are several marked differences between the Plasmodium species in terms of their worldwide distribution, life histories, and their disease pathogenesis. Further, the zoonotic emergence of Plasmodium species complicates the understanding of malaria transmission. Such differences likely involve diverse molecular adaptations of Plasmodium ookinetes to different mosquito species.
To escape the mosquito midgut, the ookinete must resist the digestive proteolytic milieu and attach to, disrupt, penetrate, and cross a physical barrier, the acellular, chitin-containing peritrophic matrix (PM), formed after blood meal ingestion (4,–7). Plasmodium-secreted chitinases, along with synergistic aspartic proteases (8), are key for ookinetes to traverse the acellular chitin-containing PM (4,–7). Plasmodium chitinases are classified as short form (Plasmodium falciparum CHT1 [PfCHT1], Plasmodium gallinaceum CHT2 [PgCHT2], and Plasmodium relictum CHT1 [PrCHT1]) and long form (PgCHT1, Plasmodium vivax CHT1 [PvCHT1], and Plasmodium berghei CHT1 [PbCHT1]) based on the presence or absence of proenzyme and the chitin-binding domain (CBD) at the N and C termini, respectively (9) (Fig. 1). It might be expected that Plasmodium ookinete-secreted chitinases differ among the two divergent clades of human-infecting Plasmodium species. Such clades have been given taxonomic status in the form of subgenera. Plasmodium vivax, Plasmodium ovale, and Plasmodium malariae belong to the Plasmodium subgenus, and P. falciparum belongs to the Laverania subgenus (10). Using available and recently augmented databases of avian and nonhuman primate Plasmodium genomes (11,–14), it is clear that nonorthologous genes encode short (avian) and long (nonhuman primate) chitinases. Hence, we hypothesize that there is an evolutionary pattern in the occurrence of chitinase genes related to ookinete-mosquito interactions, which we explore below.
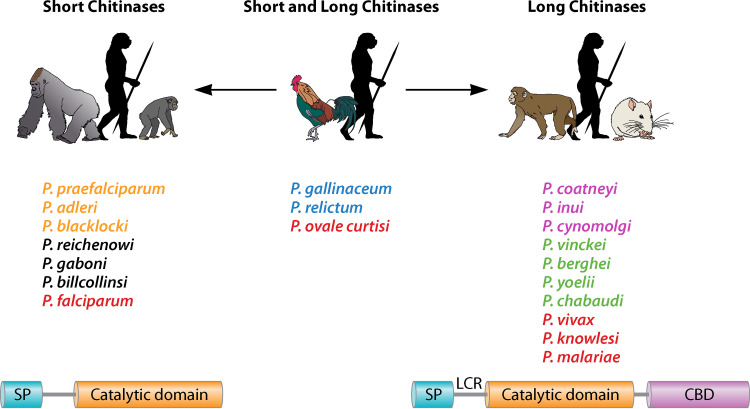
Classification of the short and long forms of chitinase found in different Plasmodium species. Long forms of chitinases have a proenzyme and putative chitin-binding domain in addition to substrate-binding and catalytic domains, whereas short-form chitinases lack proenzyme and putative chitin-binding domains. (SP, signal peptide; LCR, low-complexity region; CBD, chitin-binding domain). Plasmodium parasites infecting gorillas are shown in orange, chimpanzees in black, humans in red, birds in blue, monkeys in magenta, and rodents in green. (This figure was created with BioRender.)
With the recent availability of the curated genomic data of P. ovale curtisi and P. malariae (13), predicted amino acid sequences of chitinase were newly elucidated for these species. P. ovale curtisi is the only human (indeed, mammal)-infecting malarial parasite identified to date that contains both short and long chitinases in its genome (Fig. 2). Notably, the genome of P. malariae contains the long chitinase (ortholog of PvCHT1) and appears to have a pseudogene encoding a short chitinase, the PfCHT1 ortholog. This putative pseudogene is not assigned to a chromosome, so its annotation should be taken with caution. Nevertheless, of the two forms, the orthologs of the short chitinases are found in P. falciparum and related species (Laverania subgenus) and in P. ovale; these are shared by avian parasites (e.g., the short chitinases, PfCHT1, PoCHT1, PgCHT2, and PrCHT1). The long chitinase is found in all other Plasmodium species that infect humans, nonhuman primates, and rodents (e.g., PvCHT1, Plasmodium knowlesi CHT1 [PkCHT1], and PbCHT1) (9) and is also found in the genomes of the avian-infecting Plasmodium species sequenced to date (Fig. 1). Avian-infecting Plasmodium species contain both short and long chitinases (9). Based on this evidence, we hypothesize that the two chitinases were ancestral in Plasmodium species from mammals known today and that the two forms serve different functions (Fig. 2).
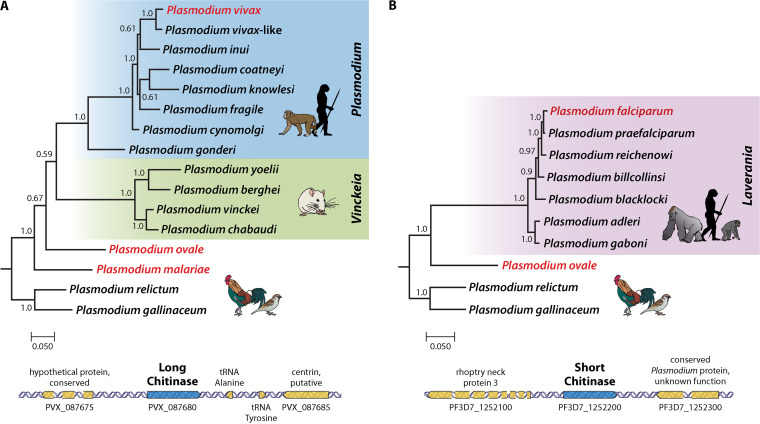
Bayesian phylogenetic hypothesis of Plasmodium parasites based on gene sequences encoding long (A) and short (B) forms of chitinase from complete parasite genomes available in PlasmoDB. The values at the nodes are posterior probabilities. Primarily human-infecting parasites are shown in red, and color bars indicate the Plasmodium subgenus.
The roles of Plasmodium ookinete-secreted chitinases in escaping from the mosquito midgut vary among Plasmodium species. The ability of both P. falciparum and P. gallinaceum to form oocysts on the midgut wall has experimentally been blocked by adding allosamidin, a specific chitinase inhibitor, to an infectious blood meal (6, 15) and by PfCHT1 gene disruption in the case of P. falciparum (16). These observations are consistent with the known maturation kinetics of P. falciparum and P. gallinaceum ookinetes, with maximal chitinase secretion at least 18 to 24h postexflagellation and -fertilization (7, 17). Furthermore, the data suggest that PM traversal is essential to avian and Laverania clade parasites, both of which have the short chitinase (7, 16). In contrast, Plasmodium berghei, whose ookinetes egress the midgut prior to PM formation (18) and which contains only a long chitinase (18), are not prevented from mosquito midgut invasion by either chitinase gene disruption or the presence of allosamidin in an infectious blood meal (18). The latter observation suggests the possibility that Plasmodium species with long chitinases might have evolved the mechanisms for early ookinete formation and midgut egress that occur prior to PM formation. This possibility merits further experimental investigation.
We recently showed that the short-form chitinases of P. falciparum (PfCHT1) and P. gallinaceum (PgCHT2) are secreted in a reduction-sensitive, high-molecular-weight (HMW) hetero-multimeric complex likely involved in mosquito midgut invasion. Proteomically identified partner micronemal proteins (von Willebrand factor A domain [vWA]-related protein [WARP] and secreted ookinete adhesive protein [SOAP]) seem to interact with short chitinases in a species-specific manner, as demonstrated by proteomic analysis of the results of chitin bead pulldown experiments (19). Heterologously expressed PfCHT1 in P. berghei, on the other hand, failed to secrete this HMW complex (19). To explicate this unique and complex, yet significant, biological phenomenon, we discuss in this minireview the possible explanations for this outcome. We also compare the primary structures of the chitinase-binding protein partners WARP and SOAP across some of the important species of Plasmodium genera and find domain structures conserved among the Plasmodium species, with disordered amino-terminal regions. The predicted SOAP structures for all the Plasmodium species are found to be highly unstructured, with poor confidence in orientation. Additionally, we discuss our new findings on the importance of the cysteines in PfCHT1-specific binding affinities for chitin. Plasmodium chitinases are multifunctional beyond simply hydrolyzing chitin, and studying their biology in detail promises to yield broad insights into Plasmodium cell biology beyond simply penetrating the PM.
PLASMODIUM CHITINASES ARE CENTRAL TO AVIAN AND SUBGENUS LAVERANIA OOKINETES’ JOURNEYS IN THE MOSQUITO MIDGUT
The PM is a physical barrier to Plasmodium invasion of the mosquito midgut, where parasites have evolved multiple mechanisms to traverse it (20). However, only a small number of ookinetes successfully swim deep into the proteolytic milieu within the mosquito midgut and cross the PM to form oocysts on the midgut wall. Molecular mechanisms driving the ookinete-to-oocyst transition remain relatively poorly studied, particularly considering the difference between Plasmodium species infecting humans. However, the composition and structure of the PM are known to be critical factors for ookinete invasion (21). The classification of the types of peritrophic membrane (types I and II) formed depends on the site of synthesis inside the host. The type I PM acting as a semipermeable membrane in dipteran vectors of Plasmodium is thought to have a key role in blood meal digestion, defending both the mosquito itself from pathogens and mechanical injury and also the parasite inside the mosquito midgut (18, 22, 23).
Importantly, the pathway of PM development is either de novo (in Aedes aegypti) or formed from the apical-end secretion vesicle in midgut epithelial cells (in Anopheles stephensi and Anopheles gambiae) (24). The natures of the structure and timing of PM formation are less well known in other mosquito species. In A. aegypti mosquitoes, PM maturation coincides with the timing of ookinete invasion, thus acting as a physical barrier to parasite invasion. In A. aegypti, the PM become evident by light and electron microscopy at 4 to 8 h after the blood meal, attains maturity and thickness by 12h, and finally forms crescentic layers by 24
h (25). Studies conducted on An. darlingi mosquitoes show that the PM is fully formed between 24 and 48
h after the blood meal, with the first appearance starting as early as 12
h, thus acting as a mechanical barrier to parasite invasion (26). Similar studies conducted on An. stephensi show the presence of compact peritrophic membranes within 30 to 72
h after the blood meal (27). Understanding the role of the PM as a physical barrier in Plasmodium infection relies on the use of the various combinations of vectors and parasites. Studies of P. falciparum and P. gallinaceum using a parasite-refractory line of An. stephensi enabled ookinete maturation but without parasite escape from the midgut lumen, suggestive of the PM being a barrier (24).
For successful transmission at the geographic and ecological levels, the parasite may have to adapt to different environments and mosquito species, including mosquito immune responses and the physiology of the mosquito midgut (28). Plasmodium-secreted chitinases have an indispensable role in the PM traversal event, as suggested by the studies conducted with allosamidin, a potent chitinase inhibitor, and with antichitinase antibodies, which prevented oocyst formation when the antibodies were fed to the mosquito in a blood meal (6, 9). The mature Plasmodium ookinete-secreted chitinases (EC 3.2.1.14) are glycosyl hydrolases that belong to the GH18 family and have been identified to play an essential role in penetrating the PM by disrupting the fibrillar structure of the PM through its chitinolytic activity (29). The GH18 family Plasmodium chitinases consist of the classical triosephosphate isomerase (β/α)8 TIM-barrel fold, with a conserved catalytic DXDXE motif known to degrade chitin (a polymer of N-acetylglucosamine [GlcNAc] and an integral part of the PM) by hydrolyzing the β‐1,4‐linkages (5). The other characterized substrate-binding motifs of P. falciparum chitinase contains the consensus sequence XXXSXGG, where X represents hydrophobic amino acids, with the exception of the nonconservative isoleucine substituted for lysine at the amino-terminal end. There is significant secondary-structural homology between the chitin-binding domain (CBD) present in the Plasmodium chitinase (long) and the CBDs of bacterial chitinases but not the CBDs of the eukaryotic chitinases (7).
Earlier characterizations of Plasmodium ookinete-secreted chitinases were done mainly with an avian parasite, P. gallinaceum. On the other hand, the studies with P. gallinaceum identified the secretion of two chitinase genes, pgcht1 and pgcht2, based on immunological and biochemical analyses (7). P. gallinaceum ookinetes secrete two separate chitinase activities with different pH optima, sensitivities to allosamidin, and molecular masses, as detectable by the antibodies recognizing the active sites of the chitinases (7). Bioinformatic analysis of the early P. falciparum genome project enabled identification of PfCHT1 (22), which was later found to be the true ortholog of PgCHT2 and structurally different from PgCHT1 (9, 12, 30). Among the Plasmodium chitinases, PgCHT1 is secreted as a proenzyme and is activated by ookinete-secreted proteases, whereas PfCHT1 and PgCHT2 are secreted in the active form (7, 22). Their varied pathways to activation during midgut invasion may be a part of an evolutionarily adapted strategy for successful mosquito midgut invasion.
Long Plasmodium chitinases have four conserved cysteine residues located in a C-terminal putative chitin-binding domain, in contrast to the very different distribution of three cysteines present in the central functional (enzymatic) domain of the short chitinases (7). To functionally characterize this domain, three conserved cysteines present in the putative CBD of PgCHT1 were mutated to serine. Mutated PgCHT1 proteins were found to interact with chitin, but their binding was more sensitive to high-percentage-detergent washes than wild-type PgCHT1 chitin binding, with the wild type being less sensitive to such treatment, demonstrating the importance of these residues in chitin binding. Despite the lack of a putative chitin-binding domain, recombinant PfCHT1 (rPfCHT1) shows specific, high-affinity chitin binding (19). Modification of conserved cysteine residues in rPfCHT1 almost completely eliminates binding to chitin beads (H. Kaur and J. M. Vinetz, unpublished data), consistent with the critical role that a pair of cysteines plays in the enzymatic and chitin-binding functions of PfCHT1 (see below). Studies conducted with a transgenically modified PfCHT1 protein, the gene for which was truncated at the nucleotides encoding the predicted C terminus of the protein (13 amino acids [aa] upstream of the stop codon), showed the essential role of the full-length protein in Anopheles freeborni mosquito midgut invasion (16). PfCHT1 disruption appeared to impair PfCHT1 secretion (16). Along with PgCHT1/2 and PfCHT1, chitinase proteins in the other human (P. vivax) and rodent (P. berghei) parasites have been studied, but at the cellular level (23). Knockout studies of the long chitinase PbCHT1 (a structural ortholog of PgCHT1) showed the nonessentiality of PbCHT1 relating to mosquito midgut invasion, likely because P. berghei ookinetes develop early and egress the midgut before full PM formation; similarly, incorporation of the chitinase inhibitor allosamidin into an infectious P. berghei bloodmeal failed to prevent oocyst formations (18).
CHITINASE-RELATED MOSQUITO MIDGUT INVASION MACHINERIES DIFFER IN DIFFERENT SPECIES OF PLASMODIUM OOKINETES
Generally, ookinetes, sporozoites, and merozoites are the three invasive stages of Plasmodium parasites known to invade different types of host cells during their life cycle (19). The sporozoites in order to achieve a productive hepatocytic infection need a well-coordinated release of the components from their secretory organelles and possess micronemes and rhoptries present at the apical end of the polarized cells for host cell invasion (31). Following the parasites’ successful initial replication in the liver (exo-erythrocytic schizogony), the parasites undergo asexual multiplication in erythrocytes (erythrocytic schizogony). The polarized merozoite invades a new red blood cell upon contact through the use of its structural components, namely, micronemes, rhoptries, and dense granules. The micronemes and rhoptries localized at the apical end of the merozoite, along with dense granules, play an essential role in erythrocyte recognition and binding, remodeling of invaded cells, and establishing the parasitophorous vacuole (PV) for the replication and formation of daughter-infecting cells. The success of this complicated process of host erythrocyte invasion requires an interplay between various proteins; some of them form an integral part of the protein complex released through merozoites in a sequential and regulated manner (31, 32). In contrast, membrane-bound micronemes appear to be the sole secretory organelle of Plasmodium ookinetes to carry out the important functions (33). This is consistent with the observation that this parasite stage does not form a PV (34), and rhoptry and dense granules are not observed (35, 36). Micronemes are trafficked to the apical complex via microtubules and release protein contents mainly during and after host cell invasion and are involved in gliding, motility, and cell traversal (35, 36).
We recently showed that PfCHT1 and PgCHT2 are secreted in a reduction-sensitive, high-molecular-weight hetero-multimeric complex likely involved in mosquito midgut invasion (19). WARP and SOAP are micronemal proteins that interact with short chitinases in a high-molecular-weight complex. These interacting protein partners, WARP and SOAP, were identified by proteomics, and there are orthologs in all Plasmodium species with genome data available (Fig. 3). Importantly, their interactions with the short chitinases seem to be affected by the parasite's genetic background. In particular, the heterologous expression of PfCHT1 in P. berghei failed to produce this HMW complex (19).
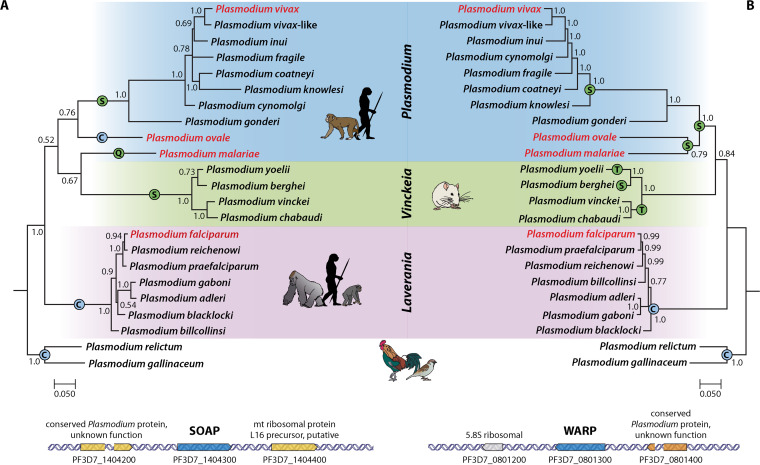
Bayesian phylogenetic hypothesis of Plasmodium parasites based on gene sequences encoding secreted ookinete adhesive protein (SOAP) (A) and von Willebrand factor A domain-related protein (WARP) (B) from complete parasite genomes available in PlasmoDB. The values at the nodes are posterior probabilities. Human-infecting parasites are shown in red, and color bars indicate the Plasmodium subgenus. Amino acid residues in the N-terminal region of WARP and SOAP are indicated on the branches or nodes.
We have identified possible structural differences to explain the inability of the P. berghei WARP and SOAP micronemal partner proteins to form a complex with the P. falciparum chitinase. Below, we show the primary structures of the chitinases and of their binding protein partners WARP and SOAP across all Plasmodium genera and found domain structure conservation with disordered amino-terminal regions. The predicted SOAP structures were found to be highly unstructured/disordered, with no predicted secondary structures, for all Plasmodium species. The highly disordered SOAP structures may be a result of the functional misfolding of the protein. This might enable the SOAP protein to function as a scaffold protein in the chitinase-containing complex. Additionally, we discuss our new findings on the importance of the cysteines in PfCHT1’s specific binding affinities for chitin.
Overall, evidence suggests that Plasmodium chitinases exhibit other functions beyond hydrolyzing chitinase as part of penetrating the PM; these functions include being secreted via micronemes in an invasion complex, binding with high affinity to chitin in the mosquito PM, and forming intermolecular complexes as part of this binding complex. Such functions differ between the major Plasmodium clades from which human malaria parasites originate. Thus, studying their biology promises to yield broad insights into Plasmodium cell biology.
MICRONEME-SECRETED HETERO-MULTIMERIC HIGH-MOLECULAR-WEIGHT PROTEIN COMPLEX COMPONENTS AND THE OOKINETE INVASION PROCESS
During evolution, apicomplexan parasites have developed different strategies for invading host cells, whether nucleated or not. Apicomplexan parasites possess a critical structure involved in the invasion process known as the apical complex, consisting of micronemes and rhoptries (37). The apical complex has evolved as a critical invasion apparatus used by most apicomplexan parasites for extracellular secretions. Electron micrograph studies of P. gallinaceum ookinetes have shown the possible secretory pathway present at the apical end of the parasite used by the parasite at the time of PM traversal (30, 36, 38). The ultrastructural details of the mature ookinete show that micronemes (the ookinete’s only secretory organelle) are trafficked by microtubules to the apical end of the parasite, making them an essential component of ookinete invasion (36, 39). The P. gallinaceum ookinetes show pores and extruded polar rings present at the apical end, which might enable PM attachment and further targeted release of the micronemal contents to initiate the invasion process by dissolving the chitinous structure (30, 36). The cargo of proteins released from micronemes enables progressive steps of invasion, motility, and ookinete-to-oocyst transition (35). Earlier studies show that the primary composition of the micronemal protein repertoire includes both soluble and transmembrane proteins (35, 40). The transmembrane proteins act as an essential component for invasive locomotion of the parasite and subsequent infection of the host (41), and soluble micronemal proteins have a putative role in the midgut invasion. A few studies have illustrated the detailed proteomic analysis of P. berghei for further understanding of the infection of the vector species using multidimensional protein identification technology (MudPIT) (42). A comparison of the proteomes of the three invasive stages, namely, merozoite, sporozoite, and ookinete, revealed the presence of some proteins specific to ookinete stages. The P. berghei ookinete’s proteomic analysis in the mosquito vector revealed the predominance of mainly surface-associated and secreted micronemal proteins, like P28, P25, circumsporozoite thrombospondin-related anonymous protein (TRAP)-related protein (CTRP), LCCL lectin domain adhesive-like proteins (LAP-1 and -2), perforin-like proteins (PLP-3 and -4), WARP, SOAP, and chitinase (42, 43). In contrast, there is a lack of proteomic data for the P. falciparum and P. vivax zygote and ookinete stage. However, the sexual-stage proteomic data obtained from P. gallinaceum using MudPIT can be extrapolated for the understanding of the mechanisms of the P. falciparum-mosquito interaction, owing to their evolutionary relatedness. The P. gallinaceum proteome data show the enrichment of CTRP and chitinase in the ookinete and secretome but not in the zygote stages (40). Also, the earlier confocal and immunoelectron studies have shown the increased concentrations of chitinases (PgCHT1 and PfCHT1) at the apical end of the ookinete associated with micronemes, which are also seen extracellularly in the midgut milieu during invasion (16, 38). A proteomic analysis of P. berghei and P. gallinaceum ookinete micronemes has demonstrated proteases, proteins with probable secretory functions, and proteins involved in parasite-vector interactions, with chitinase, SOAP, and CTRP being particularly abundant (35, 40).
Western blot data of P. gallinaceum ookinete culture supernatants have shown the presence of an HMW (>200-kDa) band (7, 17). Within this complex, WARP was identified as covalently interacting with chitinase in this reduction-sensitive HMW band fraction (7, 33). Our studies aimed to characterize the chitinase-containing HMW complex in the P. gallinaceum system and to test whether secretion of this chitinase-containing HMW complex is conserved among other Plasmodium species. Recent data confirm the involvement of the short chitinase form (both PgCHT2 and PfCHT1) in a high-molecular-weight (~1,300kDa), hetero-multimeric, reduction-sensitive complex secreted by the mature Plasmodium ookinetes. The use of a novel, highly specific chitin pulldown assay combined with de novo mass spectrometry has enabled the identification of the composition of this chitinase-containing complex (19). The main partner proteins identified were WARP and SOAP, and WARP was found to be linked to chitinases through reduction-sensitive intermolecular interactions. The stoichiometry of short chitinase WARP:SOAP remains to be determined by quantitative mass spectrometry.
The ookinete’s metabolic investment in configuring and synthesizing the native high-molecular-weight invasion complex might benefit the ookinete in midgut invasion. For a successful transitional event, a number of interactions might occur between the ligands present on the mosquito midgut surface and their corresponding extracellularly secreted ookinete proteins (44,–46). The time and level of expression of HMW complex partner proteins generally coincide with Plasmodium mosquito midgut invasion time. WARP, one of the partner proteins, consists of a single von Willebrand factor-type adhesive-module-like domain (A domain) (vWA) preceded by a signal peptide. Generally speaking, mammalian A domains are involved in cell-cell/matrix interactions, such as in platelet-collagen adhesion and function in multiprotein complexes (47). Notably, A domains are present in a number of Plasmodium proteins, including the ookinete-expressed CTRP and the sporozoite’s TRAP proteins in Plasmodium species (33, 48), suggesting a common role in adhesion as part of the invasion process. Western blot analysis of P. berghei ookinete-produced WARP after 12 h of fertilization and of recombinantly expressed PbWARP shows the presence of the protein in the large SDS-resistant oligomeric complexes (49). Similarly, megadalton SDS-resistant complexes of recombinant PfWARP have also been observed using size exclusion chromatography-multiangle light scattering when produced in the Escherichia coli system under in vitro conditions, suggestive of the high adhesive properties of the WARP protein because of functional misfolding (50) (Kaur and Vinetz, unpublished). The characteristics of the vWA domain present in WARP and the tendency to form a large oligomeric disulfide-bonded structure might enable the protein to create an adhesive molecular network between the ookinete and mosquito midgut surfaces with high-affinity binding. Another noncovalently linked protein partner identified in the chitinase-containing complex is SOAP, which is expressed mainly in ookinetes and young oocysts and secreted extracellularly by ookinetes via micronemes (51). Like WARP, SOAP is capable of forming disulfide-linked high-molecular-mass complexes which may act as multidomain adhesive molecules to participate in the midgut invasion process, like CTRP (51, 52). Also, PbSOAP, similar to orthologs of P25, P28, and CTRP, was found to interact with A. gambiae midgut component laminin γ1, thus playing a role in the ookinete-to-oocyst transition and thus mediating midgut invasion (45, 53, 54). These monomeric or homomultimeric WARP and SOAP complexes might interact with other micronemal proteins, like chitinase, through covalent and noncovalent interactions, thus holding and guiding chitinases to perform their chitinolytic activity for the degradation of PM and successful invasion. Further, investigations at the biochemical and molecular levels of this complex will provide more functional insights into ookinete invasion biology.
THE CHITINASE COMPONENT OF THE HETERO-MULTIMERIC, CHITINASE-CONTAINING, OOKINETE-SECRETED COMPLEX IS COMPRISED SOLELY OF SHORT CHITINASES
P. berghei has been the organism of choice for understanding the mosquito-stage Plasmodium biology, mainly because of its ability to be transgenically manipulated (55). However, as seen above, P. berghei and rodent-infecting, as well as non-Laverania human- and nonhuman primate-infecting, Plasmodium spp. have long chitinases (except P. ovale curtisi). Chimeric P. berghei parasite lines expressing the short chitinase, PfCHT1, was not secreted as a part of a high-molecular-weight protein complex (19). Mass spectrometry analysis performed on ookinete culture supernatants of P. berghei chimeric lines expressing PfCHT1 showed the presence of PfCHT1, PbWARP, PbSOAP, and other micronemal proteins. Questions arose as to why heterologously produced PfCHT1 was not secreted as a part of the high-molecular-weight complex. Why were P. berghei WARP and SOAP proteins functioning in a species-specific manner in this chimeric system (i.e., not interacting with PfCHT1) despite their high conservation among the orthologs (Fig. 3)? The inability of short chitinases to use the endogenous machinery of P. berghei to be part of the HMW complex may be due to their preference for their species-specific, ookinete-secreted micronemal partner proteins or, perhaps, some other reason. Both WARP and SOAP are encoded by a single-copy gene and are stage-specifically and abundantly expressed by malaria ookinetes and secreted via micronemes. WARP has shown significant cross-species conservation: the PfWARP amino acid sequence is 62% identical to that of PgWARP, and PbWARP is 61% identical (49). The carboxyl-terminal von Willebrand factor A domain contains three cysteine residues strictly conserved among the different Plasmodium WARP proteins and four cysteines within the vWA domain of the Plasmodium TRAP gene (33). However, the amino-terminal regions of all the Plasmodium WARP proteins contain various numbers of cysteine residues and do not share any similarity with the already-defined structures for proteins. Comparing the primary structures of PbWARP (long-form chitinase) and PfWARP (short-form chitinase) revealed one conserved cysteine residue in the N terminus of PfWARP instead of the serine present in PbWARP. Multiple-sequence alignment (MSA) of full-length WARP proteins for all the Plasmodium species was performed, and all species with short chitinases were found to have one conserved cysteine residue in the N-terminal structure of the WARP protein, compared to the serine/threonine present in all the Plasmodium species with the long form of chitinases (see Table S1 in the supplemental material and Fig. 1A).
Similarly, SOAP, also a cysteine-rich protein (12/13 residues), possesses two cysteine-rich domains that contain six closely placed cysteine residues (51). Among members of the Plasmodium genus, only the Laverania subgenus and avian-infecting species have a conserved cysteine residue in the region between the two conserved cysteine-rich domains. In addition to this, SOAP of the Laverania subgenus members consists of tandem repeat insertion of the 4-aa sequence glutamate‐proline‐glutamate‐valine in the less conserved region between the two domains. The MSA of the full length of SOAP revealed findings similar to those for WARP; all the Plasmodium species with the short form of chitinases have a conserved cysteine residue compared to the serine/glutamine present in Plasmodium species with the long form of chitinases (Table S1 and Fig. S1B). These residues are indicated in the WARP and SOAP phylogenies to indicate their presence across orthologous genes during the evolution of Plasmodium (Fig. 3).
The conserved cysteine of WARP and SOAP present in Plasmodium with short chitinases might be involved in the intermolecular covalent cross-linkages with the partner proteins (chitinases) responsible for the formation of the reduction-sensitive multimeric high-molecular-weight chitinase complex. However, in vitro and in vivo protein-protein interaction studies will be required to validate this fundamental yet exciting question of how the components of this species-specific HMW complex interact to carry out their functions. The other fundamental questions that remain to be answered are as follows. How do the constituent proteins get assembled in a complex and secreted through micronemes during the course of midgut invasion, and what physiological significance does this ookinete-secreted HMW complex have in the mosquito stage of the Plasmodium life cycle possessing the different forms of chitinases? Nevertheless, this complex plays an important role in ookinete invasion of the mosquito midgut. Hence, characterization of this pathway might reveal targets for malaria transmission-blocking strategies.
EVOLUTION OF ESSENTIAL MICRONEMAL PROTEINS INVOLVED IN OOKINETE INVASION
Only avian-infecting malarial parasites were considered to have two functional copies of chitinase genes, unlike the mammalian Plasmodium parasites that possess only one copy of the chitinase gene (9). The two currently available avian parasite genomes confirm the two forms of chitinase, the long and the short form (Fig. 2). However, here, we discovered that at least P. ovale also has the two genes, as do the avian-infecting parasites. The two chitinase genes were not analyzed in the same phylogenetic tree because they are not strictly orthologous. As stated above, we hypothesize that the most recent common ancestor of all known primate and rodent malaria parasites had the two forms of chitinases, so it was preserved in P. ovale and possibly in P. malariae. This hypothesis can be tested when more haemosporidian parasite genomes become available. Importantly, the separation between P. vivax and P. falciparum indicates a functional divergence between these parasites in terms of the role played by chitinases. Although it has been proposed that P. falciparum is closely related to avian-infecting malarial parasites (10, 56), its relationship with other ape parasites has long been recognized (57). Such a relationship was further demonstrated with the discovery of and whole-genome analysis of additional Laverania lineages, which include seven species infecting African great apes (58, 59). Importantly, all Laverania species have a short chitinase. Thus, losing the long chitinase in Laverania took place early in the radiation of the subgenus, whereas losing the short chitinase in the P. vivax clade seems a more recent event, considering that P. ovale kept both ancestral forms and that P. malariae at least has a pseudogene.
Although only a few Plasmodium chitinases have been functionally characterized, they cover distinct evolutionary parasite lineages: P. falciparum (short chitinase), the rodent malarias (long chitinase) or of the subgenus Vinckeia, the Plasmodium vivax clade (long chitinase), and the avian parasites represented by P. gallinaceum. Based on the data, these chitinases define how the parasite interacts with the vector. It will be interesting to explore the coevolution of micronemal proteins as they meet the demand of the Plasmodium-vector relationship. Parasites switched their vertebrate hosts, from birds to primates, and vectors, from culicine to anopheline mosquitoes, early in the radiation of Plasmodium species. During this process, some Plasmodium species lost one or the other form of chitinases. In the case of the short chitinase, its function was kept by maintaining a conserved cysteine residue in its partner proteins. It is worth noticing that several residues in each of the chitinases seem to be under strong purifying selection, as analyzed using fixed-effects likelihood (FEL) analysis, a phylogenetically based analysis to detect patterns of selection acting on the evolution of a protein-coding gene (60). This is not surprising if such proteins are functionally important, as they seem to be. The fact that the long chitinase may have a different way of interacting with other proteins during this process does not preclude its functional importance. Unfortunately, we still lack functional data from P. vivax, which might, in turn, document the role of the long chitinase in that parasite’s transmission. There is more to potentially explore regarding P. ovale, as we know that it has two genes encoding the two chitinase forms, but the fundamental importance of this is not yet understood. The pseudogenization of the short chitinase in P. malariae, if it is real, should be studied, particularly in the context of learning more about the functional aspects of the long chitinase.
AlphaFold PLASMODIUM PROTEIN STRUCTURE PREDICTIONS SHOW STRUCTURAL DIFFERENCES OF PROTEINS IN HMW-COMPLEX FORMATION
Establishing the essential three-dimensional (3D) structural framework of the Plasmodium ookinete-secreted chitinase complex protein partners provides important information on the structural-functional relationship of the proteins. Moreover, the recombinant expression of soluble and properly folded Plasmodium proteins is a challenging task, and the resistance of the P. falciparum proteins to heterologous expression has been attributed mainly to the presence of an AT-rich genome, larger protein sizes, unique glycosylation patterns, the presence of introns, and disordered structures (61). Our previous efforts to express soluble and functional forms of rPfWARP and rPfCHT1 in an E. coli system have proven the complexity of the process (Kaur and Vinetz, unpublished data). The main difficulty in expression has largely been attributed to the presence of reactive cysteines and long disordered N-terminal structures in PfWARP which might result in the production of SDS-resistant high-order oligomers. In an earlier study, Yuda et al. demonstrated the presence of PbWARP as SDS-resistant complexes produced from mature ookinetes after 12h of fertilization and from recombinantly produced PbWARP using a baculovirus-insect cell system (49). In addition to these difficulties, determining the protein structure using experimental techniques remains laborious for some complex proteins (62).
Numerous computational methods have been developed to predict protein structures from predicted primary amino acids, but their accuracy is suboptimal (63). Recently, DeepMind Technologies facilitated access to AlphaFold, an artificial intelligence network, to predict 3D protein structures de novo (64,–66). Using AlphaFold, we have predicted the protein structures for some of the important ookinete-secreted proteins involved in mosquito midgut invasion. The structure prediction scores were found to be very high for Plasmodium chitinases and WARPs of human-, rodent-, and bird-infecting Plasmodium species. We confirmed that the 3D structures of three long (PvCHT1, PbCHT1, PgCHT1) and two short (PfCHT1, PgCHT2) chitinases have a conserved triosephosphate isomerase (TIM) barrel structure consisting of eight beta strands (β1 to β8) tethered to the eight alpha helices constituting the catalytic domain of varied lengths (Fig. 4). The major structural differences among the two forms of chitinases remain with the presence/absence of the C-terminal putative chitin-binding domain (CBD). Our rPfCHT1 mutational study results are more in line with the AlphaFold 3D structural predictions for the short chitinases, which clearly show the role played by the cysteines (in PfCHT1, positions −220 and −230, and in PgCHT2, positions −219 and −229) in maintaining the properly folded and functional protein structure through the formation of intramolecular disulfide linkages (Fig. 4). The key finding is that a third conserved cysteine residue is predicted to be unbound and surface exposed, and it seems to be independent of maintaining the protein structure, with enzymatic activity and chitin-binding functions in short chitinases. Thus, it might be involved in forming the intermolecular protein-protein interactions leading to the experimentally demonstrated hetero-multimeric, reduction-sensitive, short-chitinase-containing protein complexes. In parallel, structural predictions for WARPs (other ookinete-secreted soluble proteins) are consistent with the previous finding showing the presence of a conserved vWA-like domain in all the Plasmodium species with varied amino-terminal regions (Fig. 5). The distinctive features of the WARP protein seen among the Plasmodium species with short and long chitinases are present at the N-terminal region of the protein. The Laverania subgenus species WARPs all have five cysteine residues in the large unstructured N-terminal region, compared to the four cysteines present in other species. One of the cysteine residues is conserved only within Laverania and avian-infecting Plasmodium species, not in other species, where serine (S)/threonine (T) residues are found at that orthologous position. AlphaFold predicts that this conserved cysteine is present at the N terminus, where it remains surface exposed, has potential involvement in intermolecular cross-linkages with the other binding partners, and stabilizes the entire high-molecular-weight protein complex. The only class of proteins for which AlphaFold was not able to predict a high-confidence structure was the SOAP proteins (the third ookinete-secreted soluble protein found in the complex). The predicted structures for SOAP members of all the Plasmodium species are predicted to be unstructured/disordered in their unbound state, which can be attributed to the presence of two cysteine-rich domains, with each domain having six cysteine residues. As discussed above, the one cysteine residue found in between domains II and III is conserved in the Laverania and avian-infecting Plasmodium parasites but not in other species having the long form of chitinases.
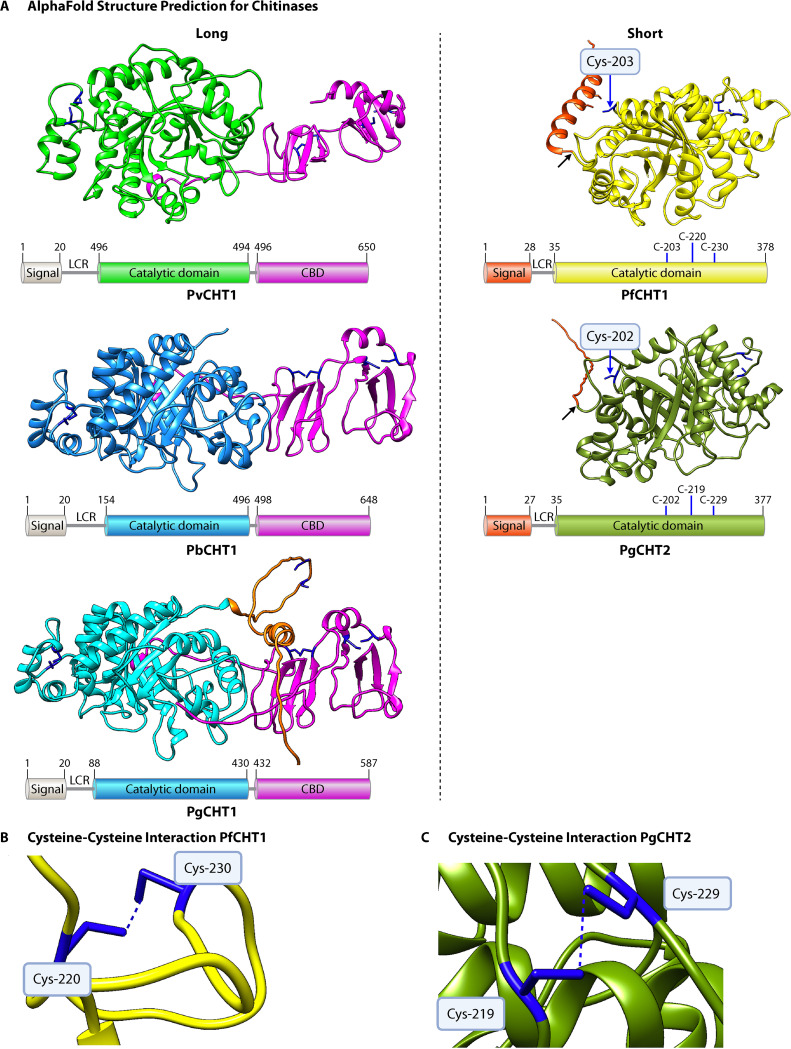
AlphaFold predictions for the structures of chitinases in P. vivax, P. berghei, P. gallinaceum, and P. falciparum. (A) Chitinases are categorized as either long or short, with the long chitinases having a chitin-binding domain. The long chitinases were trimmed to exclude the signal peptide and low-complexity regions, except that some of the low-complexity region is still included for PgCHT1. A schematic representation of long chitinases is included under the structures, showing a distinction between catalytic and chitin-binding domains. The signal peptide sequences for all the represented protein structures were predicted using SignalP 5.0 (69). For short chitinases, the three cysteines within the catalytic domain are included in the schematic, and the free, surface-exposed cysteine is labeled and marked with a blue arrow on the model. The signal peptide cleavage site is marked with a black arrow for the short chitinases. The low-complexity region is indicated on the schematics with the abbreviation LCR. (B) Closer view of the projected disulfide bridge formed between cysteines at amino acid positions 220 and 230 in PfCHT1. (C) Closer view of the projected disulfide bridge formed between cysteines at amino acid positions 219 and 229 in PgCHT2.
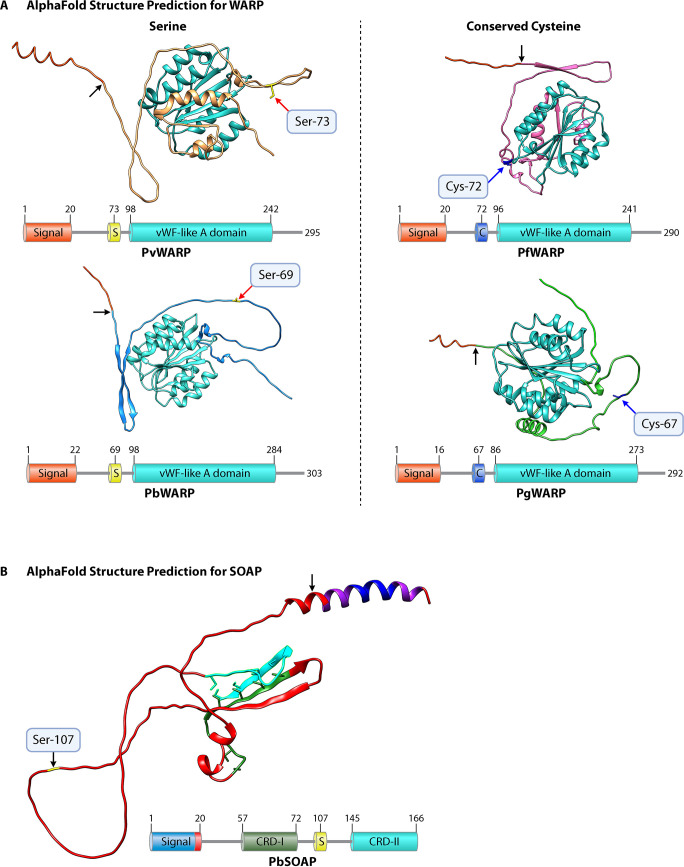
AlphaFold predictions for the structures of WARP and SOAP in P. vivax, P. berghei, P. gallinaceum, and P. falciparum. (A) Both P. vivax and P. berghei have a serine, shown in yellow, rather than a conserved cysteine, marked with a red arrow and labeled. Both P. falciparum and P. gallinaceum have a conserved cysteine, marked with a blue arrow and labeled. The signal peptide cleavage site is marked with a black arrow; the signal peptide has been trimmed in all proteins. The associated protein schematic shows functional components of protein. A conserved von Willebrand factor (vWF)-like A domain is seen across all four species, predicted using PROSITE (70), shown in turquoise. (B) "Unstructured" PbSOAP is representative of the structure prediction for SOAP for all species. The signal peptide cleavage site is marked with black arrow, and serine is shown in yellow and is present in all Plasmodium species with long chitinases (except glutamine in P. malariae). Except for the signal peptide, the structure prediction for SOAP was unconfident. The schematic shows the presence of two cysteine-rich domains (I and II) having six cysteine residues in each domain. These domains are conserved in almost all the Plasmodium species except for one cysteine residue present in between the region of the two domains in the members of only the Laverania subgenus and avian-infecting species.
Moreover, this intriguing SOAP structure leads us to rethink its function within the midgut during the PM invasion process. One hypothesis that may explain the SOAP structure is that it might be a member of the class of intrinsically disordered proteins (IDPs) (67). The IDPs usually have high conformational dynamics and flexibility with preformed sticky binding residues, such as cysteines capable of forming intermolecular covalent bonds (50). The functional misfolding of SOAP leads to sequestration of some key interactive residues inside the noninteractive areas, thus preventing the nonnative binding to SOAP, and might be involved in the regulation or signaling by complementing the functional repertoire of Plasmodium-secreted chitinase (68). Therefore, we hypothesize that the function of SOAP is to act as a scaffold protein to enable the assembly of other protein partners (chitinases and WARP) secreted by mature ookinetes and thus plays a major role in the PM traversal and midgut invasion (Fig. 6). The conservation of cysteines in SOAP and WARP proteins might have evolutionary significance that led to the unique evolution of these proteins in different Plasmodium species, specifically enabling parasite adaptation to different vector mosquitoes and their peritrophic matrices.
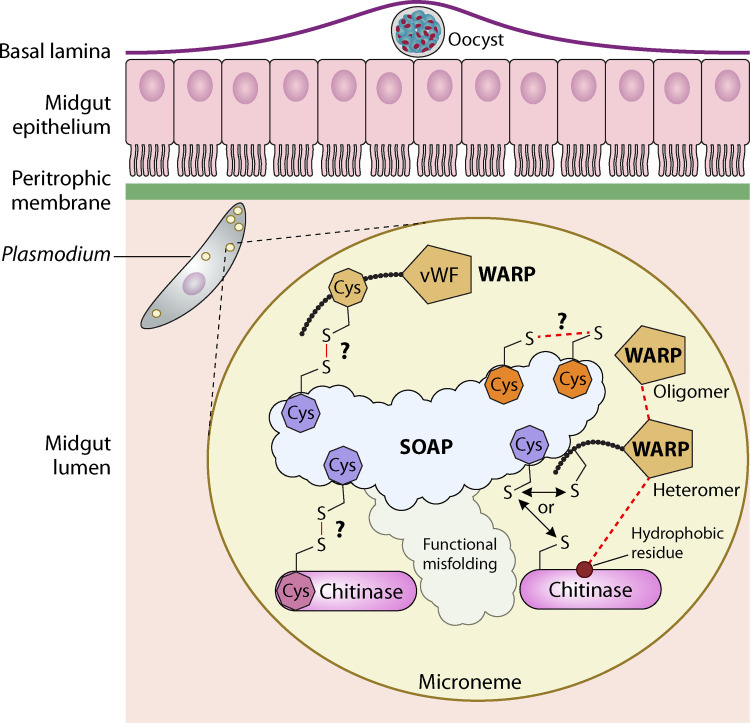
Schematic representation of the mosquito midgut invasion process where Plasmodium-secreted SOAP acts as a scaffold protein. SOAP is predicted to be unstructured/disordered for Plasmodium species. The multiple cysteine residues (dark-orange octahedral structure) present in the structure might be involved in forming intramolecular disulfide linkages. However, the conserved cysteine residue (purple octahedral structure; shown three times for better representation) in Pf/PgSOAP might be present as a free residue in the less-conserved region separating two cysteine-rich domains. The functional misfolding of the SOAP protein may prevent the nonspecific interactions of the cysteines by hiding the key elements inside nonreactive grooves and thus enable interaction with only native species-specific binding partners (chitinase and WARP) through covalent intermolecular linkages. The WARP protein forms oligomers and also heteromers by enabling its interaction with hydrophobic patches present on the chitinase surface (shown with the forward slash). The hetero-multimeric WARP-chitinase protein complex then might interact with the SOAP protein through the conserved cysteine of WARP or surface-exposed cysteine residue present in the chitinase protein for intermolecular disulfide linkages. The details about the biogenesis and localization of the hetero-multimeric complex remains to be further elucidated; this is where the biogenesis may take place as soon as the proteins are secreted from the membrane-bound microneme from the apical end of the parasite. Understanding these details will uncover fundamental aspects of Plasmodium invasion-related organelle function, with direct relevance to understanding the role of micronemal biogenesis and transport in mosquito midgut invasion. (The figure was created with BioRender.)
CONCLUSIONS
Plasmodium species with the short form of chitinases secrete the protein as a component of a reduction-sensitive, hetero-multimeric, high-molecular-weight (HMW) protein complex having SOAP and WARP as their binding partners. We predict that this ookinete-secreted invasion complex mediates PM attachment and penetration by ookinetes, particularly those species whose ookinetes develop after PM development, viz., subgenus Laverania and avian-infecting Plasmodium species. Despite the lack of a chitin-binding domain, they demonstrate strong binding affinity toward the solid substrate chitin and might enable the parasite to mediate the first step of midgut invasion via the peritrophic matrix and possibly other ligands, such as glycoconjugates involved in recognition, attachment, and invasion. During the coevolution of proteins secreted during ookinete invasion, some Plasmodium species lost one or the other form of chitinases. However, those with short chitinase have conserved cysteine residues in their partner proteins. Further structural peculiarities of the micronemal proteins SOAP and WARP might enable the parasite to have an improved function of midgut invasion. However, many functional questions still remain to be answered. Specifically, it would be interesting to identify the following.
- 1.
How have Plasmodium ookinetes’ secreted micronemal proteins functionally diverged with time?
- 2.
When and what signals are required for the packaging of proteins in the micronemes and secretion of this HMW complex during the course of midgut invasion?
- 3.
Do WARP and SOAP oligomerize first and then cross-link with chitinase to hold it together and allow chitinases to disrupt the PM focally?
- 4.
Where is the site of multimeric assembly of the invasion complex machinery?
Further understanding of chitinase-containing complex at the cellular and biochemical level will enhance our understanding of the biology of malaria transmission.
ACKNOWLEDGMENTS
We are grateful to the project managers and scientists at DeepMind, U.K., particularly Agata Laydon and Caroline Low, for their kind discussions and facilitation of the use of the AlphaFold2 algorithm to generate the structural prediction models presented herein.
We thank Adithyan Menon at Temple University for his work in browsing Plasmodium genomes to help identify genes reported here.
The work reported here was partially supported by U.S. Public Health Service grants from the National Institutes of Health, R01AI45999, R21AI053781, and U19AI089681.
Footnotes
Supplemental material is available online only.
Supplemental file 1
Supplemental material. Download IAI.00314-21-s0001.pdf, PDF file, 3.3 MB
REFERENCES
Articles from Infection and Immunity are provided here courtesy of American Society for Microbiology (ASM)
Full text links
Read article at publisher's site: https://doi.org/10.1128/iai.00314-21
Read article for free, from open access legal sources, via Unpaywall:
https://www.ncbi.nlm.nih.gov/pmc/articles/PMC8788677
Citations & impact
Impact metrics
Citations of article over time
Alternative metrics

Discover the attention surrounding your research
https://www.altmetric.com/details/114495882
Smart citations by scite.ai
Explore citation contexts and check if this article has been
supported or disputed.
https://scite.ai/reports/10.1128/iai.00314-21
Article citations
The Genome of Plasmodium gonderi: Insights into the Evolution of Human Malaria Parasites.
Genome Biol Evol, 16(2):evae027, 01 Feb 2024
Cited by: 2 articles | PMID: 38376987 | PMCID: PMC10901558
Plasmodium 6-Cysteine Proteins: Functional Diversity, Transmission-Blocking Antibodies and Structural Scaffolds.
Front Cell Infect Microbiol, 12:945924, 08 Jul 2022
Cited by: 8 articles | PMID: 35899047 | PMCID: PMC9309271
Review Free full text in Europe PMC
Why Plasmodium vivax and Plasmodium falciparum are so different? A tale of two clades and their species diversities.
Malar J, 21(1):139, 03 May 2022
Cited by: 11 articles | PMID: 35505356 | PMCID: PMC9066883
Review Free full text in Europe PMC
Structure-function analysis of cysteine residues in the plasmodium falciparum chitinase, PfCHT1.
Protein Sci, 31(5):e4289, 01 May 2022
Cited by: 1 article | PMID: 35481637 | PMCID: PMC8994504
Similar Articles
To arrive at the top five similar articles we use a word-weighted algorithm to compare words from the Title and Abstract of each citation.
A Hetero-Multimeric Chitinase-Containing Plasmodium falciparum and Plasmodium gallinaceum Ookinete-Secreted Protein Complex Involved in Mosquito Midgut Invasion.
Front Cell Infect Microbiol, 10:615343, 08 Jan 2021
Cited by: 4 articles | PMID: 33489941 | PMCID: PMC7821095
Plasmodium ookinete-secreted chitinase and parasite penetration of the mosquito peritrophic matrix.
Trends Parasitol, 17(6):269-272, 01 Jun 2001
Cited by: 39 articles | PMID: 11378031
Review
Chitinases of the avian malaria parasite Plasmodium gallinaceum, a class of enzymes necessary for parasite invasion of the mosquito midgut.
J Biol Chem, 275(14):10331-10341, 01 Apr 2000
Cited by: 68 articles | PMID: 10744721
Plasmodium ookinete-secreted proteins secreted through a common micronemal pathway are targets of blocking malaria transmission.
J Biol Chem, 279(25):26635-26644, 06 Apr 2004
Cited by: 53 articles | PMID: 15069061
Funding
Funders who supported this work.
HHS | NIH | National Institute of Allergy and Infectious Diseases (3)
Grant ID: R21AI053781
Grant ID: U19AI089681
Grant ID: R01AI45999
HHS | NIH | National Institute of Allergy and Infectious Diseases (NIAID) (1)
Grant ID: R01AI45999
NCATS NIH HHS (1)
Grant ID: UL1 TR001863
NIAID NIH HHS (3)
Grant ID: U19 AI089681
Grant ID: R21 AI053781
Grant ID: R01 AI045999