Abstract
Free full text

Use of Real‐World Data and Physiologically‐Based Pharmacokinetic Modeling to Characterize Enoxaparin Disposition in Children With Obesity
Abstract
Dosing guidance for children with obesity is often unknown despite the fact that nearly 20% of US children are classified as obese. Enoxaparin, a commonly prescribed low‐molecular‐weight heparin, is dosed based on body weight irrespective of obesity status to achieve maximum concentration within a narrow therapeutic or prophylactic target range. However, whether children with and without obesity experience equivalent enoxaparin exposure remains unclear. To address this clinical question, 2,825 anti–activated factor X (anti‐Xa) surrogate concentrations were collected from the electronic health records of 596 children, including those with obesity. Using linear mixed‐effects regression models, we observed that 4‐hour anti‐Xa concentrations were statistically significantly different in children with and without obesity, even for children with the same absolute dose (P = 0.004). To further mechanistically explore obesity‐associated differences in anti‐Xa concentration, a pediatric physiologically‐based pharmacokinetic (PBPK) model was developed in adults, and then scaled to children with and without obesity. This PBPK model incorporated binding of enoxaparin to antithrombin to form anti‐Xa and elimination via heparinase‐mediated metabolism and glomerular filtration. Following scaling, the PBPK model predicted real‐world pediatric concentrations well, with an average fold error (standard deviation of the fold error) of 0.82 (0.23) and 0.87 (0.26) in children with and without obesity, respectively. PBPK model simulations revealed that children with obesity have at most 20% higher 4‐hour anti‐Xa concentrations under recommended, total body weight–based dosing compared to children without obesity owing to reduced weight‐normalized clearance. Enoxaparin exposure was better matched across age groups and obesity status using fat‐free mass weight‐based dosing.
Enoxaparin is a low‐molecular‐weight (2,000–8,000 Da) heparin that prevents the formation of activated factor X (Xa), a serine protease that plays an essential role in the coagulation cascade. 1 Despite the fact that the US Food and Drug Administration (FDA) label states that “the safety and efficacy of [enoxaparin] in children has not been established,” enoxaparin is commonly dosed in children both with and without obesity. 1 However, obesity‐induced pharmacokinetic (PK) changes and appropriate dosing in children with obesity remains unclear. While not indicated for pediatric populations, the FDA does recommend therapeutic drug monitoring of enoxaparin via anti‐Xa concentration in certain special populations, namely adults with renal impairment or obesity, 1 yet direct measurement of enoxaparin concentration in plasma is challenging given that it is administered as a mixture of polymeric molecules with varying chain length. Therefore, measurement of anti‐Xa concentration in plasma serves as a surrogate for enoxaparin concentration, with the higher the anti‐Xa concentration, the higher the degree of anticoagulation. 2 Therapeutic drug monitoring occurs by comparing maximum anti‐Xa concentration (at 4 hours post dosing) to target ranges of 0.6–1.0 IU/mL for treatment of deep vein thrombosis with or without pulmonary embolism or 0.1–0.3 IU/mL for prophylaxis of deep vein thrombosis or ischemic complications from non–Q‐wave myocardial infarction. 1 , 3 , 4 Therapeutic drug monitoring and dose adjustment to achieve these target ranges helps to mitigate the risk of subtherapeutic or supratherapeutic concentrations (serious thromboembolic events and risk of bleeding events, respectively). 3 , 4
Patients with obesity may be at greater risk of supratherapeutic anti‐Xa exposure and, therefore, additional bleeding risk, due to weight‐based dosing coupled with obesity‐induced physiological changes that can impact enoxaparin’s PK. Recommended enoxaparin dosing from the American College of Chest Physicians (ACCP) is weight‐based (i.e., 1.0 mg/kg twice‐daily for treatment and 0.5 mg/kg twice‐daily for prophylaxis) for both children and adults. 1 , 5 This means that patients with obesity receive higher absolute doses (i.e., in milligrams) than their counterparts without obesity, which could place patients with obesity at greater risk of major bleeding events. Enoxaparin exhibits significant renal elimination (with ~ 40% of the dose excreted unchanged in urine in adults), and renal clearance is often altered with obesity due to increases in kidney size and function with obesity. 1 , 6 , 7 This increase in clearance does not increase proportionally with overall increases in total body size and absolute dose, leading to potentially higher anti‐Xa exposure. 8 , 9 , 10 , 11 Additionally, enoxaparin's volume of distribution is approximately equal to 4 L in adults, indicating that its distribution is restricted to the vasculature. 1 Consequently, higher absolute doses in patients with obesity may sequester in the blood and lead to elevated concentrations.
Enoxaparin PK studies in children with and without obesity are limited, most likely due to many ethical and logistical challenges to studying children with obesity. A stigma of obesity can further lower pediatric enrollment rates, making it challenging to include the full age and body size range of children required to characterize drug disposition. Additionally, indirect measurement of enoxaparin concentration in plasma via anti‐Xa imposes further challenges to characterizing changes to its PK with obesity. Also, many prior studies of enoxaparin rely only on concentrations taken 4 hours post dosing instead of full concentration‐vs.‐time profiles, more typical of PK studies. As a result, the objective of this study was to use real‐world data (RWD) from a multicenter electronic health record (EHR)–derived data repository developed by the Pediatric Trials Network (PTN) to evaluate differences in enoxaparin drug dosing and disposition in children with obesity using linear mixed‐effects regression models and physiologically‐based pharmacokinetic (PBPK) modeling.
METHODS
RWD source
RWD were curated from the PTN data repository, a deidentified EHR‐derived data warehouse that captures clinical information for more than 350,000 encounters in ~ 265,000 children across nine US hospitals from January 2013 to June 2017. 12 The inclusion criteria for this study included all patients 2–17 years of age receiving enoxaparin for any indication. Exclusion criteria included children with evidence of severe renal dysfunction (i.e., serum creatinine > 4 mg/dL) or hepatobiliary disease or hemolysis (i.e., bilirubin levels ≥ 6 mg/dL); children on a ventricular assist device, extracorporeal membrane oxygenation, or dialysis; and children who were pregnant, diagnosed with a neoplasm, or whose height or anti‐Xa concentration records were missing. Additional exclusions included children with only a baseline, predose anti‐Xa concentration recorded (n = 13), children with anti‐Xa concentrations recorded >80 hours after the last recorded dose (n = 18), and children with implausible height or body mass index (BMI) records attributed to recording errors (n = 45) (Table S1 ). Creatinine clearance (CLcreatinine) for observed participants in the RWD was estimated using the Bedside Schwartz equation as shown in Eq. 1 :
where height and serum creatinine are measured in centimeters and milligrams per deciliter, respectively. 13 Dosing ≤ 0.6 mg/kg was considered to be for a prophylaxis indication and >0.6 mg/kg for a treatment indication. The final data set used for analysis included 596 children with 1,099 encounters and 2,825 anti‐Xa concentrations. Full demographic and baseline data can be found in Table 1 and Tables S2 and S3 . This study was approved by the Duke University Institutional Review Board (Duke University as the coordinating center) and all participating sites with a waiver of informed consent requirements. 12
Table 1
Comparison of participant demographics, enoxaparin dosing, and anti‐Xa 4‐hour concentration
Enoxaparin dosing for treatment | |||
---|---|---|---|
Children without obesity (n = 415) | Children with obesity (n = 104) | P valuea | |
Demographics | |||
Age (years) | 9.8 (5.0) | 10.0 (5.2) | 0.73 |
Age group | |||
≥ 2 and < 6 years | 126 (30.4%) | 35 (33.7%) | 0.26 |
≥ 6 and < 12 years | 117 (28.2%) | 21 (20.2%) | |
≥ 12 years | 172 (41.4%) | 48 (46.2%) | |
Weight (kg) | 34.8 (19.5) | 58.4 (39.8) | < 0.001* |
Height (cm) | 132.4 (30.4) | 133.5 (34.7) | 0.77 |
BMI (kg/m2) | 18.0 (3.2) | 28.1 (9.1) | < 0.001* |
BMI percentile (%)b | 50.8 (29.2) | 98.0 (1.5) | < 0.001* |
Extended BMI (%) | 79.6 (10.3) | 120.1 (24.6) | < 0.001* |
Sex | |||
Male | 226 (54.5%) | 60 (57.7%) | 0.63 |
Female | 189 (45.5%) | 44 (42.3%) | |
Race | |||
White | 232 (55.9%) | 48 (46.2%) | 0.04* |
Black or African American | 102 (24.6%) | 24 (23.1%) | |
Otherc | 81 (19.5%) | 32 (30.8%) | |
Ethnicity | |||
Hispanic/Latino | 79 (19.0%) | 28 (26.9%) | 0.10 |
Not Hispanic/Latinod | 336 (80.9%) | 76 (73.1%) | |
Serum creatinine (mg/dL) | 0.49 (0.36) (10.4%) | 0.51 (0.28) (13.5%) | 0.38 |
Enoxaparin dose | |||
Weight‐based dose (mg/kg) | 1.08 (0.25) | 1.13 (0.37) | < 0.001* |
Total dose (mg) | 31.3 (17.4) | 34.3 (25.6) | < 0.001* |
Anti‐Xa concentration | |||
Concentration (IU/mL) | 0.67 (0.28) | 0.78 (0.29) | < 0.001* |
Dose‐normalized concentration (IU/mL) | 0.027 (0.019) | 0.031 (0.023) | < 0.001* |
Enoxaparin dosing for prophylaxis | |||
---|---|---|---|
Children without obesity (n = 78) | Children with obesity (n = 41) | P valuea | |
Demographics | |||
Age (years) | 11.0 (4.7) | 12.6 (4.2) | 0.06 |
Age group | |||
≥ 2 and < 6 years | 17 (21.8%) | 5 (12.2%) | 0.35 |
≥ 6 and < 12 years | 19 (24.4%) | 9 (22.0%) | |
≥ 12 years | 42 (53.8%) | 27 (65.9%) | |
Weight (kg) | 38.8 (19.0) | 82.8 (47.2) | < 0.001* |
Height (cm) | 137.5 (29.1) | 150.5 (29.7) | < 0.03* |
BMI (kg/m2) | 19.0 (3.0) | 33.2 (12.4) | < 0.001* |
BMI percentile (%)b | 60.3 (26.9) | 98.1 (1.6) | < 0.001* |
Extended BMI (%) | 80.6 (10.5) | 130.6 (40.0) | < 0.001* |
Sex | |||
Male | 42 (53.8%) | 20 (48.8%) | 0.74 |
Female | 36 (46.2%) | 21 (51.2%) | |
Race | |||
White | 37 (47.4%) | 16 (39.0%) | 0.25 |
Black or African American | 22 (28.2%) | 9 (22.0%) | |
Otherc | 19 (24.4%) | 16 (39.0%) | |
Ethnicity | |||
Hispanic/Latino | 8 (10.3%) | 14 (34.1%) | 0.003* |
Not Hispanic/Latinod | 70 (89.7%) | 27 (65.9%) | |
Serum creatinine (mg/dL) | 0.51 (0.41) (6.4%) | 0.72 (0.51) (7.3%) | < 0.01* |
Enoxaparin dose | |||
Weight‐based dose (mg/kg) | 0.57 (0.26) | 0.49 (0.25) | < 0.001* |
Total dose (mg) | 22.2 (12.9) | 29.3 (19.6) | < 0.001* |
Anti‐Xa concentration | |||
Concentration (IU/mL) | 0.43 (0.25) | 0.48 (0.30) | 0.15 |
Dose‐normalized concentration (IU/mL) | 0.022 (0.015) | 0.022 (0.021) | 0.95 |
Comparison of participant demographics, enoxaparin dosing, and anti‐Xa 4‐hour concentration for children with vs. without obesity receiving enoxaparin for treatment and prophylaxis. Summary statistics are reported as mean (standard deviation) (% missing) for continuous variables and as count (%) for categorical variables. Demographics at the time of first record during the first encounter were used to calculate descriptive statistics. Laboratory measure summary statistics were calculated using each participant’s average value across all encounters.
BMI, body mass index; CDC, US Center for Disease Control and Prevention; IQR, interquartile range; IU, international unit; LMS, lambda‐mu‐sigma.
aContinuous variables were compared using Welch’s t tests, while categorical variables were compared using Pearson’s χ2 tests. A P value < 0.05 is considered statistically significant. The results were similar when using Mann‐Whitney U/Wilcoxon rank sum tests, after testing for normality using Shapiro‐Wilk, Kolmogorov‐Smirnov, and Levene’s tests (results not shown). bBMI percentile was calculated using the CDC growth charts and LMS methodology. Note that BMI percentiles > 99% extrapolated from the CDC growth curves can misrepresent participants at the upper extreme of body size, in which case extended BMI is a more representative metric. There were 41 and 18 children with obesity receiving enoxaparin for treatment and prophylaxis, respectively, who had BMI percentiles > 99%. cFor treatment dosing, this includes 16 Asian children (9 without and 7 with obesity), 1 American Indian/Alaska Native child without obesity, 2 Native Hawaiian/Pacific Islander children without obesity, 8 children of multiple races without obesity, and 86 children (61 without and 25 with obesity) of unknown or unreported race. For prophylaxis dosing, this includes 8 Asian children (5 without and 3 with obesity), 1 American Indian/Alaska Native child without obesity, 1 Native Hawaiian/Pacific Islander child without obesity, 5 children (4 without and 1 with obesity) of multiple races, and 20 children (8 without and 12 with obesity) of unknown or unreported race. dFor treatment dosing, this includes 12 children (10 without and 2 with obesity) whose ethnicity was unknown or not reported. For prophylaxis dosing, this includes 3 children (2 without and 1 with obesity) whose ethnicity was unknown or not reported. *Statistically significant at the α = 0.05 level.
Baseline comparisons in children with and without obesity
Baseline demographics, reported laboratory measures, concomitant medications, International Classification of Diseases, Tenth Revision (ICD‐10) diagnosis codes for clotting or bleeding events, enoxaparin dosing, and anti‐Xa 4‐hour concentration were compared between children with and without obesity receiving enoxaparin for treatment and prophylaxis. Extended BMIs were used as a measure of the extent of obesity, calculated as the participant’s BMI divided by the 95th BMI percentile for a participant’s age and sex, where children with an extended BMI ≥ 100% are considered obese. This 95th BMI percentile was obtained from the standard US Centers for Disease Control and Prevention (CDC) growth charts developed from reference data from children in the 1970s, and this definition of pediatric obesity is consistent with the one used by the American Academy for Pediatrics. 14 Continuous variables were compared using Welch’s t tests, as well as Mann–Whitney U/Wilcoxon rank sum tests after testing for non‐normality using Shapiro‐Wilk, Kolmogorov‐Smirnov, and Levene's tests. Categorical variables were compared using Pearson's χ2 tests. A P value < 0.05 was considered statistically significant.
Mixed‐effects regression model development
Variables in the data set with statistically significant differences between children with and without obesity receiving enoxaparin were included in linear mixed‐effects regression models to understand which variables (including the extent of obesity) significantly account for variability in observed anti‐Xa 4‐hour concentration. Two models were developed for anti‐Xa concentrations following enoxaparin treatment and prophylaxis dosing. Regression model development was performed using R (version 4.0.3, Vienna, Austria) and RStudio (version 1.2.1335, Boston, MA) along with the “lme4,” “mice,” and “broom.mixed” packages. Since many participants had multiple anti‐Xa concentrations recorded and data was collected from multiple sites, random intercepts for each participant and site were included in the models. Approximately 4% of CLcreatinine values were missing, so we used multiple imputation and combined the estimated regression parameters across ten imputed data sets using Rubin’s Rules. 15 The final mixed‐effects models were evaluated by residual, quantile‐quantile, and predicted vs. observed concentration plots as well as root mean square error (RMSE) as shown in Eq. 2 :
where i represents an individual sample and n is the total number of samples across all participants.
PBPK model development
To further understand mechanistic drivers of anti‐Xa exposure in children with and without obesity, a PBPK model was developed and evaluated in adults, then scaled to children to predict the RWD of children with and without obesity. The PBPK model was developed in PK‐Sim and MoBi (version 8 and 9.1, respectively, Open Systems Pharmacology Suite, Open Systems Pharmacology, www.open‐systems‐pharmacology.com) using a whole‐body model. A conversion factor of 100 IU/mL anti‐Xa to 1 mg enoxaparin dose was used. 1 Subcutaneous dosing was modeled by optimizing a first‐order absorption rate constant across multiple studies of adults receiving both single and multiple doses of enoxaparin subcutaneously. 16 , 17 , 18 , 19 , 20 , 21 Data from these studies were digitized using Graph Grabber (version 2.0, Quintessa, Warrington, UK, https://www.quintessa.org/). Elimination via heparinase in plasma and renal elimination via glomerular filtration were accounted for in the model by fixing the fraction eliminated in urine to 40%, as reported in the FDA label. 1
To account for the binding of enoxaparin to its target, enoxaparin binding to antithrombin to form anti‐Xa was modeled assuming an equilibrium dissociation constant (K D) and antithrombin concentration in excess of enoxaparin's therapeutic concentration range, and the rate of unbinding was optimized using anti‐Xa concentrations digitized from studies of adults receiving intravenous enoxaparin dosing. 17 , 22
Following adult model evaluation (see next section), the adult enoxaparin PBPK model was scaled to pediatric populations 2–18 years of age. Renal clearance for simulated participants was scaled as shown in Eq. 3 :
where CLGFR,child is the child's clearance as a function of glomerular filtration rate (GFR), GFRchild is the child’s estimated GFR, GFRadult is the typical adult GFR (110 mL/minute), fu, child is the enoxaparin fraction unbound in children, fu, adult is the fraction unbound in adults, and CLGFR,adult is the adult clearance as a function of GFR. Furthermore, GFRchild was adjusted to reflect renal maturation across the population age range using a sigmoidal postmenstrual age (PMA, the summation of gestational age (GA) and postnatal age (PNA)) model using Eq. 4 (see ref. 23):
Unlike GFR, maturation of antithrombin and heparinase was not considered during scaling, as the literature suggests that antithrombin concentrations approach adult values in the first few months following birth, and ontogeny information for heparinase was not available. 24 PK‐Sim pediatric virtual populations with and without obesity include relevant physiological parameters, including changes from adults in body weight, height, organ volumes, blood flows, and tissue composition. Virtual populations including children with obesity had increased overall body weight as determined by updated BMI‐for‐age growth curves and increased lean body weight, organ volume, blood flow, and corresponding effects on clearance processes as previously described. 7
PBPK model evaluation
For each reported adult study, virtual populations of 500 virtual participants were generated based on patient demographics from the respective study to evaluate the adult model. The number of observed concentrations falling within the 90% model prediction interval was calculated, as well as the average fold error (AFE) of the median simulated concentration for all observations using Eq. 5 :
where n is the total number of samples for a particular participant (or digitized study). Because of the heterogeneous nature of EHR data, including wide variability in dose amount, frequency, and route as well as sampling time, virtual “individualized” populations were used for pediatric model simulations to predict the pediatric RWD concentrations. These virtual “individualized” populations included 500 virtual participants with demographics matched to each particular observed participant's demographics and dosing regimen. A separate virtual “individualized” population was used for each observed child in the RWD.
Dosing simulations
Once evaluated and deemed suitable to describe both adult and pediatric patients receiving enoxaparin, the pediatric PBPK model was used to simulate various dosing schemes to hypothesize appropriate dosing for children with and without obesity. First, to evaluate how absolute and weight‐normalized clearance and volume of distribution change with increasing extent of obesity, the currently recommended enoxaparin treatment dose of 1 mg/kg total body weight administered twice‐daily was evaluated using simulated populations (n = 1,000) of children with and without obesity stratified by ages 2 to < 6 years, 6 to < 12 years, and 12–18 years (Table S4 ). 7 Next, a range of enoxaparin dosing for prophylaxis (0.2–0.6 mg/kg) and for treatment (0.7–1.5 mg/kg) administered subcutaneously twice‐daily was simulated for each group using total body weight and fat‐free mass as calculated by Eqs. ( 6a ) and ( 6b ):
here FFMmales and FFMfemales are the estimated fat‐free mass for males and females, respectively. 25 Therefore, the weight‐based descriptor that leads to matched exposure between patient subgroups can be identified.
RESULTS
Baseline comparisons and regression model development
As expected, weight, BMI, BMI percentile, and extended BMI were significantly higher among children with vs. without obesity receiving enoxaparin for either treatment or prophylaxis (Table 1 ). Race significantly differed between children with and without obesity receiving enoxaparin for treatment, whereas ethnicity significantly differed across groups in children receiving enoxaparin for prophylaxis. Estimated creatinine clearance via the Bedside Schwartz equation was significantly lower in children with obesity receiving enoxaparin for both treatment and prophylaxis.
There was substantial variability in concentration across dose and time in the RWD (Figure 1 ; Figure S1 ). Children with obesity had statistically significantly higher absolute doses and anti‐Xa 4‐hour concentrations compared to those without obesity (Table 1 ). For treatment dosing, this observation was maintained even when dose‐normalizing the concentrations, indicating that the statistically significantly higher concentration is attributable to factors beyond only dosing differences between children with and without obesity.
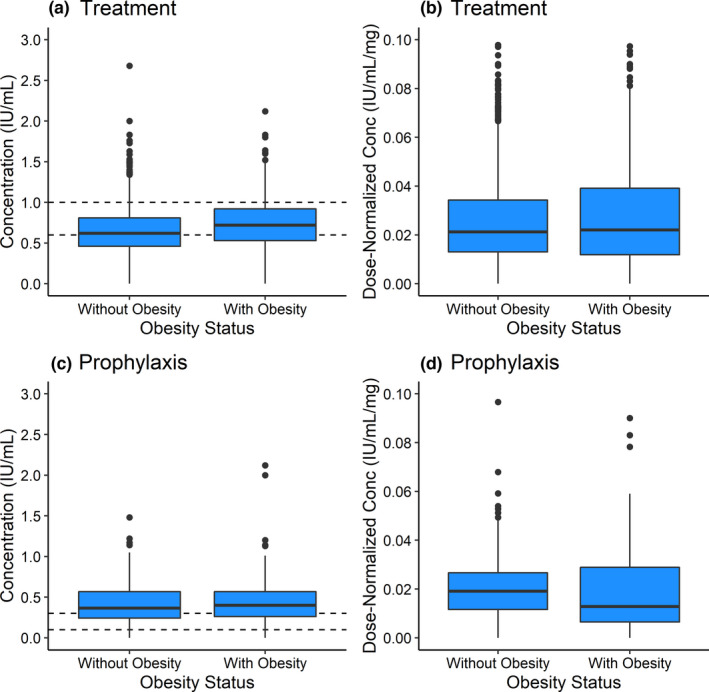
Box plots of (a, c) observed anti‐Xa concentrations and (b, d) dose‐normalized 4‐hour anti‐Xa concentrations for children with vs. without obesity receiving enoxaparin for (a, b) treatment and (c, d) prophylaxis. Dashed lines represent the target anti‐Xa concentration range for treatment and prophylaxis. The P values for comparing the median concentration in children with vs. without obesity are (a) < 0.001, (b) 0.004, (c) 0.12, and (d) 0.12. Conc, concentration.
The linear mixed‐effects regression model indicated that absolute dose, extended BMI, and the interaction between the two were significantly associated with anti‐Xa 4‐hour concentrations for participants receiving enoxaparin for treatment (Table 2 ; Figure S2 ) and prophylaxis (Table S5 ; Figure S3 ). The negative interaction term between dose and extended BMI suggested that if we compared one participant with a higher extended BMI and one with a lower extended BMI who both received the same dose, the participant with a higher extended BMI had a lower expected 4‐hour concentration. Age was statistically significant in the model for participants receiving enoxaparin for prophylaxis. Race, ethnicity, and estimated creatinine clearance were not statistically significant in either model.
Table 2
Linear mixed‐effects regression model parameters for enoxaparin treatment dosing
Parameter | Estimatea | 95% CI |
---|---|---|
Intercept (IU/mL) | 0.64 | (0.57, 0.72)* |
Absolute dose (mg) | 0.08 | (0.06, 0.10)* |
Extended BMI (%) | 0.06 | (0.03, 0.07)* |
Race – White American | −0.01 | (−0.05, 0.03) |
Race – Other Classification | −0.01 | (−0.06, 0.04) |
CLcreatinine (mL/minute/1.73 m2) | −0.01 | (−0.03, 0.004) |
Absolute dose * Extended BMI | −0.02 | (−0.03, −0.01)* |
Parameters for a linear mixed‐effects regression model regressing anti‐Xa 4‐hour concentration onto key variables for children receiving enoxaparin for treatment.
BMI, body mass index; CI, confidence interval; CLcreatinine, creatinine clearance.
aVariables were centered on the median value and scaled by the standard deviation. A random slope was fitted for each participant and site. Missing CLcreatinine values were imputed using a predictive mean matching multiple imputation method. *Statistically significant at the α = 0.05 level.
PBPK model development and evaluation
Final enoxaparin PBPK model parameters can be found in Table 3 . The PBPK model was able to capture the digitized adult literature data, with an overall AFE of 0.59 (range: 0.30–1.11) across two intravenous and eight subcutaneous single‐dose and multidose studies of enoxaparin (Table S6 ; Figure S4 ). 16 , 17 , 18 , 19 , 20 , 21 , 22 The PK across the range of dosing in these studies (20–120 mg) was linear, with predicted clearance and volume of distribution similar to reported values (Table S7 ).
Table 3
Parameters used in enoxaparin PBPK model development
Parameter | Enoxaparin | Source |
---|---|---|
Physicochemical properties | ||
Units of anti‐Xa per 1 mg enoxaparin (IU/mL) | 100 | FDA label 1 |
Molecular weight (g/mol) | 4,500 | FDA label 1 |
pKa value | 3.00 | Wang et al. 48 |
Lipophilicity | −10.0 | Drug Bank |
Solubility (mg/mL) | 200 | Drug Bank |
Blood to plasma ratio | 0.85 | Calculated valuea |
Binding | ||
Antithrombin plasma concentration (μM)b | 25 | Wajima et al. 44 |
K D (μM)b | 2.5 | Wajima et al. 44 |
k off (1/hour) | 2 | Optimized |
Distribution | ||
Partition coefficients | PK‐Sim Standard | Willmann et al. 49 , 50 |
Cellular permeabilities | PK‐Sim Standard | Willmann et al. 49 , 50 |
k a (1/hour) | 0.60 | Optimized |
Metabolism | ||
Heparinase | ||
CLspec (1/minute)c | 0.096 | Optimized |
Excretion | ||
GFR fractiond | 1.00 | FDA label 1 |
f e,urine | 40% | FDA label 1 |
CLspec, specific clearance; FDA, US Food and Drug Administration; f e,urine, fraction of dose excreted in urine; k a, absorption rate constant; GFR, glomerular filtration rate; IU, international unit; K D, equilibrium dissociation constant; k off, rate of unbinding; PBPK, physiologically‐based pharmacokinetic; pKa, negative log of the acid dissociation constant.
a
For children without obesity in the data set, the AFE (standard deviation of the fold error) was 0.87 (0.26), with 75.2% of concentrations falling within the 90% model prediction interval (20.6% above, 4.2% below). The results for children with obesity were similar, with an AFE (standard deviation of the fold error) of 0.82 (0.23) and 77.2% of concentrations falling within the 90% model prediction interval (20.5% above, 2.4% below). In a subgroup analysis, children with overweight (BMI percentile ≥ 85% to < 95%) and severe obesity (BMI percentile ≥ 99%) had an AFE of 0.79 and 0.72, respectively. There were no obvious trends in AFE with obesity status, indication, age, sex, or race (Figure S5 ). The coefficient of variation in simulated 4‐hour dose‐normalized concentration was 173.9%, slightly more than the variation of 137.6% observed for 4‐hour concentration in the RWD.
Dosing simulations
Simulated children with obesity demonstrated higher median absolute clearance (13.9%, 18.5%, and 14.3% for children 2 to < 6, 6 to < 12, and 12–18 years, respectively) and volume of distribution (19.7%, 23.7%, and 24.4% for children 2 to < 6, 6 to < 12, and 12–18 years, respectively) as compared to children without obesity (Figure 2 ). Conversely, median weight‐normalized clearance and volume of distribution was lower with obesity by a similar magnitude. Increases in absolute clearance attributable to increased kidney volume (and consequently GFR) in children with obesity did not increase to the same degree as body weight; therefore, weight‐normalized clearance decreased, as previously suggested. 7 , 26
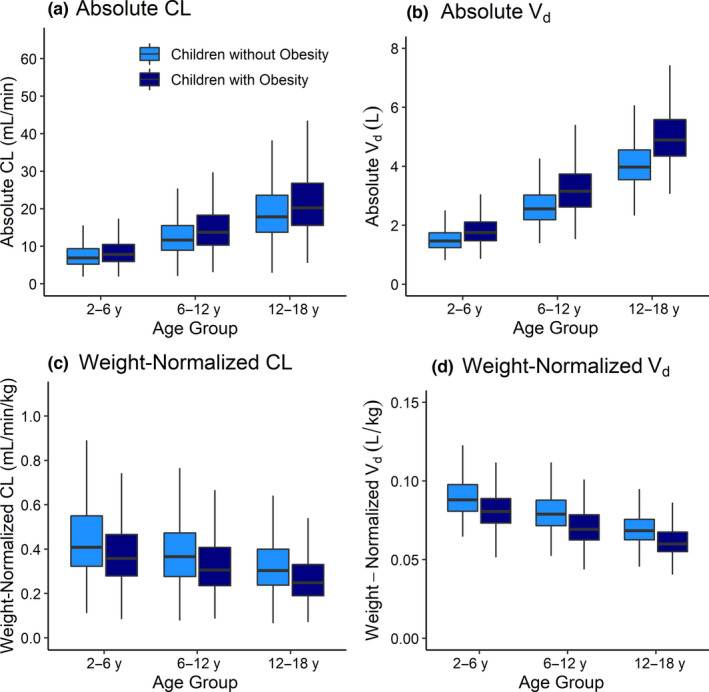
Changes in simulated enoxaparin absolute and weight‐normalized (a, c) CL and (b, d) V d with obesity status for children ages 2 to < 6 years, 6 to < 12 years, and 12–18 years with and without obesity. Simulated children (n = 1,000 per group) received 1 mg/kg subcutaneous doses twice‐daily of enoxaparin. Boxes represent the median and IQR, and whiskers extend to 1.5 × IQR. CL, clearance; IQR, interquartile range; V d, volume of distribution.
For total body weight–based dosing, there were observable differences in the simulated 4‐hour concentration, particularly between simulated children with and without obesity (Table 4 ). We observed that simulated children 12–18 years of age demonstrated the highest anti‐Xa 4‐hour concentration increase with obesity, with median 4‐hour concentrations ~ 20% higher in children with vs. without obesity using recommended treatment dosing (1.0 mg/kg). To further explore possible dosing regimens for this age group (12–18 years) using total body weight as the body composition metric, a full range of prophylactic and treatment dosing was simulated (Figure 3 ). From these simulations, simulated children with obesity in this age group required 0.3 mg/kg less dose (administered twice‐daily) than children without obesity to achieve target therapeutic concentrations. Across all dosing regimens, younger children demonstrated lower simulated 4‐hour concentrations compared with older age groups (Figure 3 ; Figures S6 and S7 ). In contrast, using fat‐free mass rather than total body weight for calculating treatment and prophylaxis doses resulted in simulated anti‐Xa 4‐hour concentration ranges that were very similar across obesity status and age subgroups of simulated children.
Table 4
Summary of PBPK model‐simulated anti‐Xa concentrations
Simulated 4‐hour anti‐Xa concentration (IU/mL) following recommended dosing for treatment (1.0 mg/kg twice‐daily) 5 | |||
---|---|---|---|
2 to < 6 years | 6 to < 12 years | 12–18 years | |
TBW‐based dosing | |||
Children without obesity | 0.52 (0.39, 0.65) | 0.59 (0.46, 0.76) | 0.71 (0.55, 0.88) |
Children with obesity | 0.60 (0.47, 0.75) | 0.70 (0.54, 0.88) | 0.86 (0.66, 1.08) |
FFM‐based dosing | |||
Children without Obesity | 0.41 (0.31, 0.52) | 0.46 (0.36, 0.58) | 0.50 (0.37, 0.62) |
Children with obesity | 0.40 (0.31, 0.61) | 0.48 (0.37, 0.61) | 0.56 (0.42, 0.72) |
Simulated 4‐hour anti‐Xa concentration (IU/mL) following recommended dosing for prophylaxis (0.5 mg/kg twice‐daily) 5 | |||
---|---|---|---|
2 to < 6 years | 6 < 12 years | 12–18 years | |
TBW‐based dosing | |||
Children without obesity | 0.27 (0.20, 0.33) | 0.30 (0.24, 0.39) | 0.36 (0.28, 0.45) |
Children with obesity | 0.30 (0.24, 0.45) | 0.36 (0.28, 0.45) | 0.44 (0.34, 0.56) |
FFM‐based dosing | |||
Children without obesity | 0.21 (0.16, 0.26) | 0.23 (0.18, 0.30) | 0.27 (0.20, 0.33) |
Children with obesity | 0.21 (0.16, 0.27) | 0.24 (0.19, 0.31) | 0.28 (0.21, 0.37) |
Summary of PBPK model‐simulated anti‐Xa concentrations following twice‐daily recommended dosing for enoxaparin for treatment and prophylaxis. Values shown as median (IQR).
FFM, fat‐free mass; IQR, interquartile range; IU, international unit; PBPK, physiologically‐based pharmacokinetic modeling; TBW, total body weight.
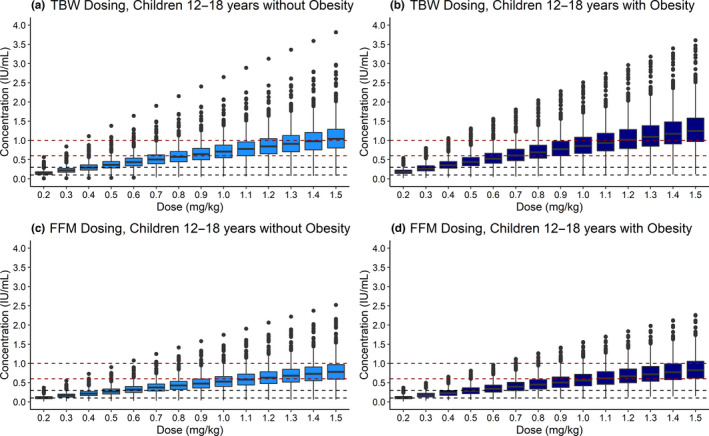
PBPK model‐simulated anti‐Xa 4‐hour concentrations following twice‐daily subcutaneous dosing of 0.2–1.5 mg/kg using (a, b) TBW or (c, d) FFM for children ages 12–18 years (a, c) without and (b, d) with obesity (n = 1,000 children per group). A full range of dosing was simulated to assess both treatment and prophylaxis target therapeutic concentration ranges. Boxes represent the median and IQR, and whiskers extend to 1.5 × IQR. Red and black dashed lines represent the target ranges for treatment (0.6–1.0 IU/mL) and prophylaxis (0.1–0.3 IU/mL) dosing, respectively. 3 , 4 Similar plots for children 2 to < 6 and 6 to < 12 years are presented in Figures S6 and S7 . FFM, fat‐free mass; IQR, interquartile range; IU, international unit; PBPK, physiologically‐based pharmacokinetic; TBW, total body weight.
DISCUSSION
This study used RWD from children with and without obesity receiving enoxaparin and found that obesity status was a significant predictor of anti‐Xa 4‐hour concentration. To better characterize this effect mechanistically, we developed a novel enoxaparin PBPK model in adults, scaled and evaluated the model in children, and then used the model to simulate appropriate dosing in children with and without obesity. While the impact of obesity on enoxaparin PK and dosing has been evaluated in adults, there have been limited studies to date in children, and none with data this extensive in the full pediatric age range in children both with and without obesity. 8 , 9 , 21 , 27 , 28 , 29 , 30 , 31 , 32 , 33 , 34 , 35 , 36 To date, this is the first PBPK model of enoxaparin, and, to our knowledge, the first PBPK model evaluated using EHR data from a national multicenter data repository, which could enable a new data source for this vital modeling tool.
Previous studies evaluating the potentially increased risk of supratherapeutic exposure in patients with obesity are conflicting for both adults and children. Some studies in adults support the recommended weight‐based dosing, suggesting that a minority of adults with obesity receive reduced healthcare provider‐adjusted dosing, and that there is no significant difference in peak concentration or bleeding events in patients with vs. without obesity. 9 , 21 , 30 , 31 , 32 , 33 Conversely, other studies support conservative dosing in adults with obesity, showing that reduced, lean body weight, and fat‐free mass dosing achieved more concentrations within the target range with reduced incidence of bleeding. 8 , 10 , 27 , 34 , 35 , 36 Although there have been limited studies in children with obesity, the results to date are similarly conflicting. One study in 30 adolescents with obesity and overweight found that reduced (< 0.9 mg/kg) and recommended (1.0 mg/kg) dosing achieved equivalent concentrations with no adverse outcomes. 28 Conversely, a study of 60 children 2–18 years of age found that the mean enoxaparin dose following routine therapeutic drug monitoring was 26% lower and concentrations were 21% higher in children with obesity, suggesting a reduced dose is more appropriate for children with obesity. 29 The results presented herein support the previous findings from the latter pediatric study.
Linear mixed‐effects regression modeling highlighted the importance of both dose and obesity status (measured by extended BMI) as significant predictors of anti‐Xa 4‐hour concentration. The observed interaction between dose and obesity status suggests that absolute doses at higher extended BMIs are associated with an increase in the anti‐Xa concentration of a lower magnitude than at lower extended BMIs. This finding makes sense within the context of known PK changes with obesity. Participants with obesity have increased kidney size and increased absolute clearance, meaning they are better able to eliminate enoxaparin renally. 7 The observed absolute creatinine clearance in the data set was ~ 10% higher for children with vs. without obesity (87.4 vs. 76.7 mL/minute for those receiving enoxaparin for treatment). However, because this increase in enoxaparin absolute clearance does not increase proportionally with body size and absolute dose, children with obesity receive higher enoxaparin doses and have higher anti‐Xa concentrations. The regression models demonstrated reduced predictive performance at the upper and lower end of observed anti‐Xa concentrations. This reduced predictive performance occurred at least partially because there were no observed samples from children without obesity at the upper end of the dose range (i.e., > 110 mg) and no children with obesity at the lower end of the absolute dose range (i.e., < 5 mg). These scenarios were not unexpected, since enoxaparin is dosed by total body weight. Therefore, these mixed‐effects model results likely only hold for the middle ~ 80% of the observed data. Other analyses, such as PBPK modeling, are needed to enable an understanding of the interaction between dosing, environmental, and demographic factors, and to extrapolate beyond this range. While a previous mixed‐effects regression analysis has not yet been conducted in children, two analyses of adults with obesity also found that body size (measured as total body weight) was a statistically significant, independent predictor of anti‐Xa 4‐hour concentration. 37 , 38
In developing the novel enoxaparin PBPK model, enoxaparin binding to antithrombin to form the anti‐Xa complex was key to evaluating the model, since enoxaparin is measured via its surrogate anti‐Xa concentration. Limited studies available in the literature reported a K D of 0.64–177 nM, with studies varying considerably in the molecular weight and affinity of heparin used for analysis. 39 , 40 , 41 , 42 , 43 This is well below enoxaparin concentrations in plasma at therapeutic doses. However, enoxaparin is widely used clinically because it is known to have dose‐linear PK, so this binding process was forced within the linear binding range by using a KD value of 2.5 μM, just above enoxaparin's therapeutic concentration range. Plasma antithrombin concentration was assumed in excess of this therapeutic concentration to allow for adequate enoxaparin binding, as previously assumed in a coagulation cascade systems model. 44 Additionally, no differences in the absorption rate constant were implemented with obesity. While increased fat mass may decrease the rate at which a subcutaneously dosed drug can reach the bloodstream, no differences in absorption rate were observed when attempting to optimize this parameter in children without vs. with obesity.
Under these assumptions, the enoxaparin PBPK model captured observed adult data and observed pediatric concentrations, with a bias toward underestimation. However, the majority of this underestimation occurred in samples taken after the last dose, as 74.9% of observed pediatric concentrations that were sampled at ~ 4 hours had an AFE of 0.94, with 79.8% of observed concentrations within the 90% model prediction interval. Adult simulated intravenous clearance (~ 24 mL/minute; Table S7 ) was similar to the FDA label‐reported adult clearance following an intravenous dose (26 mL/minute). 1 Because enoxaparin’s bioavailability in the PBPK model was nearly 100% as reported by adult studies, the simulated subcutaneous adult clearance was ~ 24 mL/minute as well, though this overestimates the FDA label‐reported subcutaneous clearance of 15 mL/minute. 1 , 17 , 22 No reports of pediatric subcutaneous clearance were identified. The pediatric volume of distribution (median 2.8 L) approximated simulated pediatric participants’ plasma volume given age as expected from enoxaparin's binding and mechanism of action. 1 Peak simulated anti‐Xa concentrations were similar to a previous report in children 12–18 years (median 0.77 vs. 0.7 IU/mL) and children ~ 6–18 years (median 0.71 vs. 0.67 IU/mL) with obesity under equivalent dosing. 28 , 29
PBPK‐model simulated lower anti‐Xa 4‐hour concentrations for younger children is in line with prior population PK analyses in children without obesity, noting a trend of decreased weight‐normalized clearance with increasing age, with allometrically scaled weight as a significant covariate. 45 , 46 This makes sense, as younger children have higher weight‐normalized clearance compared with older children. Using total body weight–based dosing, model simulations show ~ 20% higher anti‐Xa exposure for older children with obesity ages 12–18 years, potentially necessitating reduced total body weight–based dosing in older children with obesity as has been previously suggested for treatment and prophylactic dosing. 29 , 47 These results suggest that to effectively select an initial total body weight–based enoxaparin dose for children, both the child's age and body size should be considered. Alternatively, fat‐free mass‐simulated dosing resulted in similar anti‐Xa 4‐hour concentrations across all age and obesity status subgroups. Since fat‐free mass already incorporates age and body size, a fat‐free mass dosing strategy could offer a simplistic and more precise method of dose selection for physicians, provided the fat‐free mass metric can be calculated.
While this study uses extensive EHR data to address a key clinical question in children with obesity, some limitations exist. EHR data served as a valuable, extensive data source, but inherently had a large amount of variability that translated to wide variability in model simulations (due to variability in data recording, assay variability between sites, etc.). There were missing and incomplete data present within the EHR. ICD‐10 codes were used to extract diagnoses, and information on organ dysfunction may be biased, as these codes are intended primarily for billing vs. research purposes. These codes are inconsistently recorded and might be incomplete or inaccurate. Additionally, measurement techniques and accuracy of equipment for measuring weight and height cannot be standardized. The ICD‐10 codes cannot delineate with certainty which participants received enoxaparin for treatment vs. prophylaxis, so this was assumed based on initial dosing. Note that there could be other reasons for a participant to receive a low initial dose of enoxaparin apart from prophylaxis (e.g., high bleeding risk). While the data are extensive, in many cases there was only one anti‐Xa sample available per dose. EHR data collection aimed to exclude children who were critically ill to avoid confounding factors on PK comparison (e.g., children on dialysis or with severe renal and hepatic dysfunction), but observed concentrations were obtained from hospitalized children who may have other disease state factors not accounted for within the PBPK model. The observed concentrations were mostly (74.9%) within 3–5 hours post dosing, and PBPK model predictions may slightly underestimate observed concentrations. Nevertheless, this study sheds further light on the appropriate dosing of enoxaparin, a commonly used drug, in an underserved patient population of children with obesity. This study also demonstrates the utility of EHR data for PBPK model analysis, provided the sample size is large enough to overcome inherent data noise.
In conclusion, extensive RWD were used to demonstrate that obesity is a significant predictor of anti‐Xa 4‐hour concentration in children. A novel PBPK model of enoxaparin was developed to further mechanistically explore the role of obesity on anti‐Xa exposure in children. The model was able to capture observed anti‐Xa concentrations from children with and without obesity. PBPK model simulations suggest higher absolute clearance and volume of distribution in children with vs. without obesity, leading to ~ 20% higher anti‐Xa exposure in children with obesity aged 12–18 years compared with those without obesity using recommended weight‐based dosing. However, using fat‐free mass instead of total body weight under recommended weight‐based dosing resulted in more comparable 4‐hour anti‐Xa exposure across all ages and obesity classes.
FUNDING
This research was funded by the Eunice Kennedy Shriver National Institute of Child Health and Human Development (NICHD) under award 5R01HD096435. The enoxaparin pediatric data were collected by the Pediatric Trials Network (PTN) through NICHD contract HHSN275201000003I (Principal Investigator (PI): Daniel K. Benjamin, Jr.). J.G.G. received research support from a National Institute of General Medical Sciences (NIGMS) funded T32 program (T32GM122741), a Fred Eshelman Pre‐Doctoral Fellowship in Pharmaceutical Sciences from the American Foundation for Pharmaceutical Education (AFPE), and a PharmAlliance travel award. F.O.C. was funded through a UNC/GSK Pharmacokinetics/Pharmacodynamics Post‐Doctoral Fellowship. C.P.H. receives salary support for research from the NICHD (R13HD102136; RL1HD107784), the National Heart Lung and Blood Institute (NHLBI) (R61/R33HL147833), the US Food and Drug Administration (R01‐FD006099, PI Laughon; and U18‐FD006298), the US government for his work in pediatric clinical pharmacology (Government Contract HHSN275201800003I, PI: Benjamin under the Best Pharmaceuticals for Children Act), the non‐profit Burroughs Wellcome Fund, and other sponsors for drug development in adults and children (https://dcri.org/about‐us/conflict‐of‐interest/). D.G. received research support from the NICHD (5R01HD096435‐04 and 1R01HD102949‐01A1). The content is solely the authors' responsibility and does not necessarily represent the official views of the National Institutes of Health.
CONFLICTS OF INTEREST
D.G. receives research support from Nabriva Therapeutics through a contract with the University of North Carolina at Chapel Hill. In addition, D.G. serves as a consultant for Tellus Therapeutics, focusing on neonatal drug development. All other coauthors declared no competing interests for this work.
AUTHOR CONTRIBUTIONS
J.G.G., F.O.C., M.S.L., C.R.L., A.N.E., J.S., K.R.K., C.M.K., C.P.H., and D.G. wrote the manuscript. J.G.G., F.O.C., M.S.L., A.N.E., J.S., and D.G. designed the research. J.G.G., F.O.C., M.S.L., C.R.L., A.N.E., J.S., K.R.K., C.M.K., C.P.H., and D.G. performed the research. J.G.G. and F.O.C. analyzed the data.
ACKNOWLEDGMENTS
Pediatric Trials Network (PTN) Steering Committee Members: Daniel K. Benjamin Jr, Christoph Hornik, Kanecia Zimmerman, Phyllis Kennel, and Rose Beci, Duke Clinical Research Institute, Durham, NC; Chi Dang Hornik, Duke University Medical Center, Durham, NC; Gregory L. Kearns, Texas Christian University and UNTHSC School of Medicine, Fort Worth, TX; Matthew Laughon, The University of North Carolina at Chapel Hill, Chapel Hill, NC; Ian M. Paul, Penn State College of Medicine, Hershey, PA; Janice Sullivan, University of Louisville, Louisville, KY; Kelly Wade, Children's Hospital of Philadelphia, Philadelphia, PA; Paula Delmore, Wichita Medical Research and Education Foundation, Wichita, KS. The Eunice Kennedy Shriver National Institute of Child Health and Human Development (NICHD): Perdita Taylor‐Zapata and June Lee. PTN Publications Committee: Chaired by Thomas Green, Ann & Robert H. Lurie Children's Hospital of Chicago, Chicago, IL. The PTN Data Repository sites principal investigator (study team) are as follows: Andrew Atz (Leslie Lenert, John Clark, and Kalyan Chundru), Medical University of South Carolina, Charleston, SC, USA; Catherine Bendel (Brian Harvey and Sonya Grillo), University of Minnesota, Minneapolis, MN, USA; Francis Chan (Stephanie Fan), Lorma Linda University, Lorma Linda, CA, USA; Kevin Downes and Robert Grundmeier (Mark Ramos and Shawn O’Connor), Children’s Hospital of Philadelphia, Philadelphia, PA, USA; Benjamin Fogel, Penn State University, University Park, PA, USA; Debbie Gipson (Samara Attala, Richard Eickstadt, Erin Kaleba, Don Liamini, Jamie Estill, Jeremy Jared, and Peter Bow), University of Michigan, Ann Arbor, MI, USA; Matthew Laughon (Jennifer Talbert and Cindy Clark), University of North Carolina at Chapel Hill, Chapel Hill, NC, USA; Michael Miller and William Muller, Ann and Robert H. Lurie Children’s Hospital of Chicago, Chicago, IL, USA; Michael Smith (Janice Sullivan, Steve Heilman, KP Singh, Satish Vuyyuri, Jeff Schwitters, and Don Stone), University of Louisville and Norton Healthcare, Louisville, KY, USA. Erin Campbell, MS, reviewed and submitted this manuscript. Erin Campbell did not receive compensation for her contributions, apart from her employment at the institution where this study was conducted.
Contributor Information
the Best Pharmaceuticals for Children Act – Pediatric Trials Network Steering Committee:
Full text links
Read article at publisher's site: https://doi.org/10.1002/cpt.2618
Read article for free, from open access legal sources, via Unpaywall:
https://www.ncbi.nlm.nih.gov/pmc/articles/PMC9504927
Citations & impact
Impact metrics
Citations of article over time
Alternative metrics

Discover the attention surrounding your research
https://www.altmetric.com/details/129240694
Smart citations by scite.ai
Explore citation contexts and check if this article has been
supported or disputed.
https://scite.ai/reports/10.1002/cpt.2618
Article citations
Characterizing Enoxaparin's Population Pharmacokinetics to Guide Dose Individualization in the Pediatric Population.
Clin Pharmacokinet, 63(7):999-1014, 02 Jul 2024
Cited by: 0 articles | PMID: 38955947
Physiologically-based pharmacokinetic modeling of pantoprazole to evaluate the role of CYP2C19 genetic variation and obesity in the pediatric population.
CPT Pharmacometrics Syst Pharmacol, 13(8):1394-1408, 04 Jun 2024
Cited by: 1 article | PMID: 38837864 | PMCID: PMC11330186
Virtual twins for model-informed precision dosing of clozapine in patients with treatment-resistant schizophrenia.
CPT Pharmacometrics Syst Pharmacol, 13(3):424-436, 19 Jan 2024
Cited by: 1 article | PMID: 38243630 | PMCID: PMC10941576
Anti-Obesity and Anti-Inflammatory Synergistic Effects of Green Tea Catechins and Citrus β-Cryptoxanthin Ingestion in Obese Mice.
Int J Mol Sci, 24(8):7054, 11 Apr 2023
Cited by: 7 articles | PMID: 37108217 | PMCID: PMC10138730
Perioperative Acetaminophen Dosing in Obese Children.
Children (Basel), 10(4):625, 27 Mar 2023
Cited by: 0 articles | PMID: 37189874 | PMCID: PMC10136941
Review Free full text in Europe PMC
Go to all (7) article citations
Similar Articles
To arrive at the top five similar articles we use a word-weighted algorithm to compare words from the Title and Abstract of each citation.
Optimal dosing of enoxaparin in overweight and obese children.
Br J Clin Pharmacol, 88(12):5348-5358, 27 Jul 2022
Cited by: 4 articles | PMID: 35816401 | PMCID: PMC9795990
Weight-Based Enoxaparin for Venous Thromboembolism in Obesity Gives Similar Anti-Xa Levels to Patients <100 kg, with No Increase in Major Bleeding.
Semin Thromb Hemost, 45(1):94-99, 10 Jan 2019
Cited by: 3 articles | PMID: 30630208
Cancer-Associated Venous Thromboembolism Treatment With Anti-Xa Versus Weight-Based Enoxaparin: A Retrospective Evaluation of Safety and Efficacy.
Ann Pharmacother, 55(9):1120-1126, 16 Jan 2021
Cited by: 1 article | PMID: 33455432
Review of current evidence available for guiding optimal Enoxaparin prophylactic dosing strategies in obese patients-Actual Weight-based vs Fixed.
Crit Rev Oncol Hematol, 113:191-194, 22 Mar 2017
Cited by: 9 articles | PMID: 28427508
Review
Funding
Funders who supported this work.
FDA HHS (2)
Grant ID: U18 FD006298
Grant ID: R01 FD006099
NHLBI NIH HHS (2)
Grant ID: R33 HL147833
Grant ID: R61 HL147833
NICHD NIH HHS (2)
Grant ID: R01 HD102949
Grant ID: R01 HD096435
NIGMS NIH HHS (1)
Grant ID: T32 GM122741