Abstract
Free full text

Pharmacology of Signaling Induced by Dopamine D1-Like Receptor Activation
Abstract
Dopamine D1-like receptors consisting of D1 and D5 subtypes are intimately implicated in dopaminergic regulation of fundamental neurophysiologic processes such as mood, motivation, cognitive function, and motor activity. Upon stimulation, D1-like receptors initiate signal transduction cascades that are mediated through adenylyl cyclase or phosphoinositide metabolism, with subsequent enhancement of multiple downstream kinase cascades. The latter actions propagate and further amplify the receptor signals, thus predisposing D1-like receptors to multifaceted interactions with various other mediators and receptor systems. The adenylyl cyclase response to dopamine or selective D1-like receptor agonists is reliably associated with the D1 subtype, while emerging evidence indicates that the phosphoinositide responses in native brain tissues may be preferentially mediated through stimulation of the D5 receptor. Besides classic coupling of each receptor subtype to specific G proteins, additional biophysical models are advanced in attempts to account for differential subcellular distribution, heteromolecular oligomerization, and activity-dependent selectivity of the receptors. It is expected that significant advances in understanding of dopamine neurobiology will emerge from current and anticipated studies directed at uncovering the molecular mechanisms of D5 coupling to phosphoinositide signaling, the structural features that might enhance pharmacological selectivity for D5 versus D1 subtypes, the mechanism by which dopamine may modulate phosphoinositide synthesis, the contributions of the various responsive signal mediators to D1 or D5 interactions with D2-like receptors, and the spectrum of dopaminergic functions that may be attributed to each receptor subtype and signaling pathway.
1. INTRODUCTION
1.1 Background and Objective
Dopamine is a catecholamine neurotransmitter that is extensively distributed in the brain and certain peripheral organs of numerous species. In brain, neurons employing dopamine as their primary neurotransmitter innervate a wide array of nuclei, including the caudate-putamen, nucleus accumbens, lateral septum, amygdaloid complex, hippocampus, prefrontal cortex, and anterior pituitary (Nieuwenhuys, 1985; Roth et al., 1987). Among these brain regions, dopamine regulates fundamental neurobehavioral functions that range from memory and motivation to motor activity and neuroendocrine integration (Horn et al., 1979; Fluckiger et al., 1987; Jimerson, 1987; Losonczy et al., 1987). The physiological effects of dopamine are produced through its interaction with a family of dopaminergic receptors belonging to the superfamily of G protein-coupled receptors (GPCRs). Early functional studies led to the recognition of two distinct classes of these dopaminergic receptors that were then classified as D-1 and D-2 receptors (Garau et al., 1978; Kebabian and Calne, 1979; Stoof and Kebabian, 1981; Stoof and Kebabian, 1982; Onali et al., 1984). More recently, molecular cloning approaches have enabled the identification of several distinct members of the dopamine receptor family, resulting in the currently recognized subfamilies of D1-like and D2-like receptors. At the minimum, mammalian D2-like receptors comprise the D2, D3, and D4 subtypes along with their multiple splice variants and polymorphic forms (Seeman, 1980; Sibley et al., 1993; Seeman and Van Tol, 1994), whereas the D1-like subfamily includes the human D1 and D5 (or the equivalent rodent D1A and D1B) receptors (Seeman, 1980; Sibley et al., 1993; Seeman and Van Tol, 1994).
The aim of this review is to critically summarize recent research relating to the signaling systems engaged by members of the D1-like receptor subfamily, and including analytical comments on the regulatory mechanisms that might be involved in physiologically orchestrating the relative activities of these signaling systems. Further down, some speculations are presented that, if well taken, might seed new research ideas on the topic. To the extent that pharmacological tools capable of distinguishing among the D1-like receptors are yet emerging, much of the inferences and discussion will inextricably include both the D1 and D5 (and perhaps additional) subtypes of D1-like receptors. Nevertheless, in some instances, it should be possible to ascribe certain properties or responses to either receptor subtype based on findings from studies employing specific molecular manipulations or selective cellular expression of the receptors. As a convention, we will use D1-like to include any or all of the D1-like clones, while nomenclatures such as D1/D1A, D5/D1B, or D1C will be used where specific information on that subtype is available and warranted by the context. Additionally, unless otherwise indicated, the discussion will focus on the mammalian species, seeing these organisms are most commonly used as experimental and disease models and as such have yielded most available functional data.
Any attempt to clarify dopaminergic signaling and attendant physiological function is definitely a formidable task, even where the range of actions has been narrowed as in the present focus on D1-like receptors and immediate signaling responses. Thus, the goal was not for the review to be a comprehensive treatise, but to be comprehensible. Ultimately, the hope is to help paint a picture that might hint at how multiple signaling cascades could integrate to co-regulate the many functions and dysfunctions of dopamine.
1.2. Subtypes and Phylogenetic Expression of D1-like receptors
The D1 receptor was defined as a functional entity and characterized pharmacologically through the early work of Spano and colleagues (Spano et al., 1978), Kebabian and Calne (Kebabian and Calne, 1979), and others. As a molecular entity, however, it was not until the 1990s that the first member of the D1-like subfamily of dopamine receptors was isolated almost concurrently by four different groups and named as the human D1 receptor (Dearry et al., 1990; Sunahara et al., 1990; Grandy et al., 1990; Zhou et al., 1990) or the rodent D1A receptor (Zhou et al., 1990; Monsma, Jr. et al., 1990). An additional D1-like receptor was subsequently cloned in humans and termed D5 (Grandy et al., 1991), while its counterpart in the rat was named D1B (Tiberi et al., 1991). Afterwards, there was a spate of activity leading to the uncovering of multiple D1-like transcripts in different organisms. Thus, current evidence indicates that D1-like receptors are expressed in diverse species, including the fruit fly (Gotzes et al., 1994; Sugamori et al., 1995; Han et al., 1996; Reale et al., 1997; Schetz et al., 2003; Kehren and Baumann, 2005), cockroach (Orr et al., 1987; Evans et al., 1991; Evans and Green, 1991), locust (Homberg, 2002; Keating and Orchard, 2004), spider (Schmidt et al., 1981; Sauer et al., 1994), eel (Cardinaud et al., 1997), earthworm (Gardner and Cashin, 1975; Shpakov et al., 2008), flatworm (Venturini et al., 1989), goldfish (Mora-Ferrer et al., 1999), tree frog, (Agui et al., 1988; Schutte, 1991; Liu and Lasater, 1994; Behrens and Wagner, 1995) lizard (Clark et al., 2000), chicken (Agui et al., 1988; Demchyshyn et al., 1995; Schnabel et al., 1997; Sun and Reiner, 2000; Soares et al., 2000; Kubrusly et al., 2007), rodent (Grilli et al., 1988; Nisoli et al., 1988; Tiberi et al., 1991; Bryson et al., 1992; Mannoury la Cour et al., 2007), monkey (Besson et al., 1988; Sedvall et al., 1991; Choi et al., 1995; Bergson et al., 1995a; Bergson et al., 1995b) and humans (De Keyser et al., 1988a; De Keyser et al., 1988b; Dearry et al., 1990; Sunahara et al., 1990; Sidhu and Fishman, 1990; Grandy et al., 1990; Weinshank et al., 1991; Ferreira-de-Almeida et al., 1993). Among these species, multiple structurally divergent subtypes of D1-like receptors have been detected, including at least 3 transcripts in Xenopus (D1A, D1B and D1C), 4 in the chicken (D1A, D1B, D1C and D1D), 2 in the mouse or rat (D1A and D1B), 2 in the monkey, and 2 in humans (D1 and D5). Categorization of these proteins as D1-like receptors is generally deduced from structural homologies among the transcripts, pharmacological interaction with selective dopamine D1-like receptor ligands, and functional coupling to distinct stimulatory G-proteins and signaling cascades.
1.3. Peripheral and Brain Regional Distribution of D1-like Receptors
At peripheral tissues of rodents or primates, D1-like receptors are expressed in the heart (Ozono et al., 1997), in the walls of systemic arteries (Emilien et al., 1999), and in renal microvessels and proximal tubules (Girbes et al., 1992; Nash et al., 1993; Yao et al., 1998). Among these tissues, there are variations in the expression of D1/D1A and D5/D1B receptors. For instance, whereas the receptors in renal proximal tubules are of the D1 type, those in systemic arteries and renal microvasculature appear to be of the D5 subtype. How these differences in tissue distribution of the receptor subtypes may relate to the regulation of kidney function is not yet clearly understood.
Various mammalian brain regions show substantial but variable expression of D1-like receptors. Based on immunodetection techniques, D1/D1A receptors are significantly expressed in the striatum, olfactory bulb, cerebral cortex, and to a lesser extent in the hippocampus and amygdala (Levey et al., 1993; Ariano and Sibley, 1994; Bergson et al., 1995b). On the other hand, the D5/D1B receptor in the rat or monkey shows a much more widespread expression across most brain regions, including the hippocampus, amygdala, frontal cortex, striatum, basal forebrain, thalamus, hypothalamus, cerebellum and brainstem (Bergson et al., 1995b; Ciliax et al., 2000). When both receptor subtypes are considered, the immunohistochemical data correlate generally well with the mRNA and receptor binding data. Curiously, though, there is a paucity of D1-like receptor mRNA in the entopenducular nucleus, globus pallidus and substantia nigra pars reticulata of the rat, even though these regions demonstrate binding sites for D1-like receptor ligands (Mengod et al., 1991). Thus, D1A receptors, which have been specifically studied in these areas, may be synthesized elsewhere and then transported here, hence their confinement mostly to neuronal projections (Bergson et al., 1995b). Overall, the widespread distribution of D5 receptors as opposed to the more confined presence of D1 receptors in the basal ganglia suggests that these two members of the D1-like subfamily could subserve nonidentical spectra of physiological functions.
1.4. Brain Cellular and Subcellular Localization of D1-like Receptors
As with other GPCRs, D1-like receptors are synthesized in the cell cytoplasm and are then subsequently transported to the plasma membrane of cell bodies and dendrites (Hersch et al., 1994; Liu and Lasater, 1994; Caille et al., 1996). Ultrastructural visualization studies on cortical tissues demonstrate the localization of D1-like receptors in dendritic spines of medium spiny neurons as well as cortical pyramidal cells (Smiley et al., 1994; Hersch et al., 1995; Bergson et al., 1995b; Sesack et al., 2003). An extensive study by Bergson and colleagues (Bergson et al., 1995b) examined the regional, cellular and subcellular distribution of both D1-like receptors in the primate brain. At the cellular level, medium spiny neurons of the neostriatum in primates showed immunoreactivity to both D1 and D5 antibodies, although the latter occurred to a lesser extent. Conversely, the large aspiny neurons which are typically cholinergic interneurons appeared to express only D5 receptors. Differential localization of both receptor subtypes has also been clearly demonstrated in caudate and mesencephalic neuronal cell types. The largely separate localization of D1 and D5 receptors in medium sized spiny neuronal cells of the striatum has been suggested to arise either on account of variations in gene expression of each receptor subtype or as a result of differences in vesicular transport of the proteins (Bergson et al., 1995b). In cerebrocortical pyramidal cells, however, there is profuse reactivity to both subtypes of D1-like receptors and experiments involving dual labeling with both subtypes show them to be commonly co-localized in cells within each region.
Whether expressed separately or in the same cells, D1 and D5 receptors are known to show distinct patterns of subcellular distribution (Bergson et al., 1995b). Generally, D5 receptors are localized to neuronal perikarya and proximal dendrites, and occasionally in the neuropil of some tissues such as olfactory bulb, islands of Calleja, cerebral cortex, superior colliculus, and molecular layer of cerebellum (Ciliax et al., 2000). The D1 receptors on the other hand are found mostly in axon terminals and dendrites (Bergson et al., 1995b). For individual cortical pyramidal neurons, D1 receptors are expressed to a considerably higher degree in dendritic spines as opposed to the more prominent presence of D5 receptors in dendritic shafts (Bergson et al., 1995b; Muly, III et al., 1998). Thus, D1 and D5 receptors are differentially expressed among brain tissues and demonstrate distinguishable patterns of subcellular distribution that probably reflect differences in signaling and physiological roles anticipated for each subtype.
1.5. Comparative Structural Elements of D1-like Receptors
D1-like receptors in various species comprise between 445-488 amino acids with significant structural homologies among members within or between species (Dearry et al., 1990; Sunahara et al., 1991; Demchyshyn et al., 1995). D1-like receptors do not contain introns in their protein coding regions (Sunahara et al., 1990; Civelli et al., 1993; O’Dowd, 1993; Gingrich and Caron, 1993), hence there is diminished likelihood of obtaining receptor variants from these genes. Two related pseudogenes for the D5 receptor, however, have been identified on human chromosomes 1 and 2, locations that are separate from chromosomes 5 and 4 which respectively contain the genes coding for the full-length D1 and D5 receptors (Weinshank et al., 1991; Grandy et al., 1991; Nguyen et al., 1991a; Nguyen et al., 1991b; Takahashi et al., 1992; Grandy et al., 1992). The pseudogenes code for incomplete forms of the receptor which have 154 amino acids as opposed to the regular 477 found in the full length D5 receptor. These shorter peptides are expressed at lower levels and may actually serve a role different from the regular length variety. Given the emerging understanding of the significant roles played by inhibitory short RNAs in cellular function, there is a need to re-examine the possibility that these receptor pseudogenes (or “pseudogenes” in general) may participate in regulating the expression or function of the corresponding full-length transcripts.
Human D1-like dopamine receptors are up to 79% identical in their putative transmembrane domains whereas they are only 40 - 45 % similar to their siblings in the D2-like subclass. Further, D1-like receptors have potential glycosylation sites in their first extracytoplasmic loop, as well as having longer carboxyl terminal tails and shorter third intracellular loops compared to D2-like receptors (Hartman and Civelli, 1997). While differences in length and sequence composition of the intracellular loops of D1-like and D2-like receptors probably relate to the respective coupling of these receptors to stimulatory and inhibitory G-proteins (Civelli et al., 1993; O’Dowd, 1993; Gingrich and Caron, 1993), there has been less detailed exploration of the relationship between the variable cytoplasmic loops of D1-like receptor proteins and possible differences in the functional coupling of the receptors.
D1-like dopamine receptors exhibit substantial amino acid sequence conservation particularly in their transmembrane domains (Probst et al., 1992). As these domains are considered the primary sites of ligand recognition, the high sequence homology among the proteins most likely contributes significantly to the similarity in overall pharmacological profiles exhibited by D1 and D5 receptors. Further, both members of the D1-like receptor family show substantial similarities in their general protein folding patterns; this factor physiologically influences ligand recognition and receptor-effector coupling, and pharmacologically increases the difficulty of identifying drugs that functionally differentiate between the receptors (Jaber et al., 1996).
Notwithstanding their extensive similarities, there are notable differences among the D1-like receptors. The D5 receptor appears to exhibit higher affinity for dopamine than the D1 receptor when transiently or stably expressed in COS-7 or Ltk- cells (Jarvie et al., 1993). More recently, Jiang and colleagues (Jiang et al., 2005) reported on a number of compounds isolated from nature that exhibit different affinities for the D1 and D5 dopamine receptors. For instance, SBG492, one of the phytochemicals screened, exhibited an EC50 of 343 μg/ml at the D1 receptor while the value for the D5 receptor was 32 μg/ml. Photoaffinity labelling studies in transfected GH4C1 rat pituitary cells revealed the ability of photoactivated [125I]MAB, a selective D1 receptor photoaffinity radioligand, to incorporate into the D1 but not the D5 receptor; this effect was apparently independent of the particular cell line in which the receptors were expressed (Niznik et al., 1988; Sidhu et al., 1998a). Despite the structural similarity of the [125I]MAB binding sites for both receptor subtypes, critical differences between the subtypes in the protein domains around these binding sites have been suggested (Sidhu et al., 1998a). One might suspect that such differences in structural features of the D1-like receptors, coupled with their differential regional, cellular, and subcellular distribution highlighted above, would presage differences in receptor signaling and/or biological function.
2. SIGNALING CASCADES ASSOCIATED WITH D1-LIKE RECEPTOR STIMULATION
2.1. Multiplicity of D1-like Receptor Signaling Responses
Receptor signaling processes convey information from the extracellular environment across the cell membrane, or from one intracellular compartment to another, thereby leading to changes in cellular function. The information may be of a chemical, electromagnetic, or mechanical nature, and typically there are changes in the nature or amount of the information as it flows through the series of reactions constituting the signaling cascade. Neurotransmitter receptors generally signal through either metabotropic or ionotropic cascades. Ionotropic modes involve direct or molecular transducer-mediated coupling of the receptor to the operation of ion channels that regulate the flow of cations or anions into or out of the cell. There is some evidence that dopamine D1-like receptor agonists modulate ion fluxes in some tissues, although direct receptor coupling has not been definitively demonstrated. Metabotropic signaling, however, involves the enzymatic conversion of a substrate into a second messenger molecule, which then sets off a cascade of events that ultimately produce the physiological response to receptor activation. Most dopamine receptors demonstrate metabotropic coupling in native tissues as well as in transfected systems.
As members of the superfamily of GPCRs, dopamine D1-like receptors couple to cellular signaling cascades by means of a G protein which serves as the signal transducer for the receptor. In various peripheral tissues, brain regions, and transfected cell lines, dopamine is known to induce the activation of multiple G proteins and signaling cascades in a manner consistent with activation of D1-like receptors. There is, thus, extensive evidence that dopamine D1-like receptors couple to multiple metabotropic signaling cascades, some of which are in turn capable of initiating ionotropic signaling events in a variety of cell types and tissues (Undie and Friedman, 1990b; Undie et al., 1994; Bergson et al., 2003; Zeng et al., 2003; Zhang et al., 2005; Zhen et al., 2005).
Current impressions about D1-like receptor coupling mechanisms have been reached through interpretation of data derived from a mix of experimental approaches. In some studies, pharmacological agonists or antagonists with declared selectivity for D1-like receptors are tested and the observed effects attributed to D1-like receptor signaling. In other studies, the expression or functionality of specific receptor subtypes is manipulated either in native physiological tissues or by transfection into cells that do not naturally express the receptors; subsequent functional effects observed with dopamine or D1-like agonists are then attributed to D1-like receptor mediation. Each approach has its strengths and limitations that should be considered while evaluating the significance or relevance of the experimental results. For instance, the use of native tissues where the receptor is naturally expressed has the strength of physiological relevance but the weakness that other co-existing receptors may modulate the experimental observations. Conversely, using artificial expression systems affords specificity of ligand/receptor effects, but may suffer from the fact that GPCRs frequently resort to promiscuous transducer/effector coupling upon expression in artificial cell lines.
2.2. Signaling via Adenylyl Cyclase
Adenylyl cyclase (AC) is a 12-transmembrane-spanning protein that catalyzes the conversion of adenosine triphosphate (ATP) to the intracellular second messenger cyclic-3′,5′-adenosine monophosphate (cyclic AMP). The activity of AC is regulated by Gs-type G proteins, notably Gs and Golf, and these G proteins are in turn regulated by ligand-induced receptor activation. Dopamine stimulates AC activity in various dopamine-innervated tissues in the brain or peripheral sites. Indeed, it was the discovery of dopamine-sensitive AC stimulation that led to the identification and subsequent definition of the “D1” (in contrast to the “D2”) dopamine receptor. As shown in figure 1, the stimulatory effect of the “D1” receptor on AC activity was found to be conveniently counterbalanced by an inhibitory effect mediated through activation of the “D2” receptor. The introduction of this schema provided a significant intellectual boost to the conceptualization and interpretation of experimental studies on dopamine neurobiology and pharmacology.
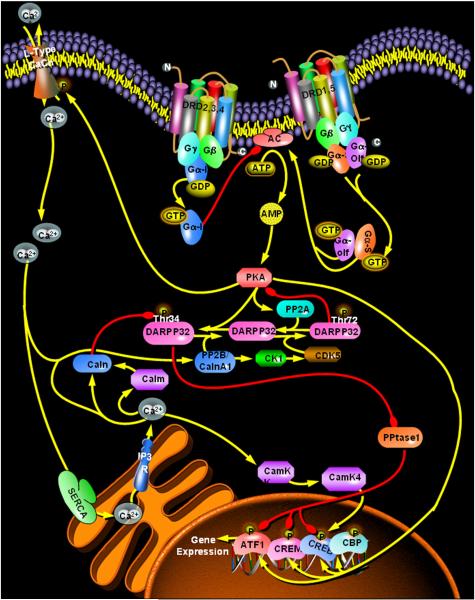
Essentials of dopamine receptor coupling to adenylyl cyclase signaling. Stimulation of D1 dopamine receptors activates the G-protein Gs, or Golf, which activates adenylyl cyclase (AC) to convert ATP into the second messenger cyclic AMP (cAMP). The D2-like receptors couple through Gi to inhibit AC, resulting in decreased production of cAMP. The primary target of cAMP is protein kinase A (PKA). PKA phosphorylates and activates the transcription factors CREB (cAMP response element-binding protein), CREM (cAMP response element modulator) and ATF1 (activating transcription factor-1). PKA-mediated phosphorylation of DARPP32 converts the latter into a potent inhibitor of protein phosphatase-1 (PPtase1). Given that PPtase1 dephosphorylates, and therefore inhibits, ATF1, CREB, and CREM, the net effect of PPtase1 inhibition is to further enhance PKA-sensitive transcriptional activation. Conversely, PKA phosphorylates the L-type calcium channel, leading to increased cytosolic Ca2+, calcium-induced Ca2+ release from the endoplasmic reticulum, and activation of calmodulin (Calm) and protein phosphatase 2B/calcineurin A1 (PP2B/CalnA1). Calm and PP2B inhibit Thr34 activation of DARPP32, ultimately counteracting the transcriptional activating effect of PKA. This Ca2+- dependent effect provides opportunity for signaling crosstalk of the dopamine-PKA system with various calcium mobilizing receptors. Note that Ca2+ activation of PP2B also results in activation of casein kinase-1 (CK1), which in turn facilitates cyclin-dependent kinase-5 (CDK5) activation. As illustrated, CDK5 participates in DARPP32 regulation, thus underlining its role in diverse dopaminergic functions. Other abbreviations: SERCA (sarcoplasmic reticulum Ca2+- ATPase), IP3R (inositol trisphosphate receptor), CamK (calmodulin-dependent kinase).
2.2.1. Receptor-mediated generation of cyclic AMP
In most dopamine-innervated tissues, stimulation of D1 receptors activates the alpha subunit of Gs which then stimulates the activity of AC leading to increased production of intracellular cyclic AMP (see Figure 1). Dopamine induces AC activity in diverse regions of the brain, notably the striatum and frontal cortex, whereas other tissues such as the hippocampus and amygdala are much less responsive (Montague et al., 2001; Jin et al., 2001; Leonard et al., 2003). While an effect of dopamine is evident in brain slices or in whole cultured brain cells, agonist potencies and efficacies are greater in membrane preparations. For instance, in whole cells, the EC50 for dopamine activation of cyclic AMP may be as much as 5.0 μM, while the EC50 in membrane preparations is in the range of (11 nM to 3.0 μM) (Blumberg et al., 1985; Tong et al., 2001). Maximal effects of dopamine are usually in the range of 7-10 fold in whole cells (Demchyshyn et al., 1995; Sugamori et al., 1998) and up to 60 fold in brain membrane preparations (Carenzi et al., 1975; Memo et al., 1983; Gilmore et al., 1995). These maximal effects are attained at drug concentrations of 30-300 uM in brain slices or whole cells (Andringa et al., 1999) and 10-100 uM in brain membranes (Carenzi et al., 1975; Memo et al., 1983; Gilmore et al., 1995). Another technical factor that may influence apparent drug responses relates to the method used for quantifying the reaction product and for calculating the pharmacological efficacy. Conducting the assay in an accumulation mode where an inhibitor of phosphodiesterase is included to block the breakdown of cyclic AMP would yield higher net effects of the test drug. Alternatively, exclusion of a phosphodiesterase inhibitor permits an analysis of the rate of formation of cyclic AMP as a function of the duration of drug exposure; with this approach the observed drug effect would depend not only on the receptor affinity and intrinsic activity of the drug, but also on the onset of action – a rather flexible term that reflects how quickly the drug can elicit a desired effect.
A wide range of dopamine D1-like agonists mimic the effects of dopamine on cyclic AMP formation. These range from the prototypic benzazepine, SKF38393, to various phenanthridine and isochroman derivatives. While compounds such as SKF38393 and SKF75670 are partial agonists in that they achieve significantly less than 100% of the efficacy of the endogenous neurotransmitter, a series of benzazepine (SKF80723, SKF82958) and isochroman (A77636, A68930) derivatives have shown full or supra-maximal (greater-than-dopamine) efficacies in stimulating cyclic AMP formation; a number of these compounds are also more potent than dopamine (Andersen et al., 1987; Andersen and Jansen, 1990; Ghosh et al., 1996). On the other hand, the effects of dopamine or of the synthetic agonists are sensitive to blockade by SCH23390, the prototypical D1-like receptor antagonist. Hence, a dopaminergic effect that is mimicked by SKF38393 and inhibited by SCH23390 is generally considered a D1-like receptor effect.
Each of the cloned mammalian D1-like receptors has been shown to couple to cyclic AMP production in transfected cells. Similarly, D1-like receptors isolated from other organisms have also shown the ability to couple to cyclic AMP production upon transfection into suitable cell lines. Thus, it has often been generalized that all D1-like receptors couple to cyclic AMP signaling, although, as would be evident in later sections, there are observations on other signaling cascades that must still be explained if this were the case (or the only case).
In stimulating cyclic AMP formation, dopamine seems to show significantly greater potency at the D1B receptor than at the D1A receptor, although this may not necessarily apply to the synthetic D1-like agonists or experiments with tissues where the receptor is natively or physiologically expressed. Moreover, the D1B receptor exhibits constitutive activation of cyclic AMP formation when expressed in diverse cell lines (Tiberi and Caron, 1994; Charpentier et al., 1996; Demchyshyn et al., 2000). While it is evident that dopaminergic coupling to cyclic AMP production is mediated via D1-like receptors, it is less clear how each of the two mammalian D1-like receptors contributes to the observed responses in native tissues. Animals bearing inactivated or deleted receptors have helped somewhat in addressing the native response issue. For example, brain tissues from D1A-knockout mice lose the ability to respond to agonist stimulation of cyclic AMP, even though the D5 receptors are apparently unaffected by the mutation (Friedman et al., 1997). Conversely, tissues from D5 knockout animals continue to show significant D1-like agonist stimulation of cyclic AMP. Hence, following D1-like agonist stimulation in native brain tissues, most of the cyclic AMP response observed is probably mediated through the D1A receptor. This would imply that the D5 receptor, notwithstanding its widespread expression, contributes little to the regulation of dopamine signaling and brain function, at least to the extent that such regulation depends on AC signaling. To the contrary, D5 receptor knockout mice show behavioural changes that differ from the effects of D1A receptor knockout. Hence, as an alternative explanation to the loss of cyclic AMP response in D1A knockout mice, perhaps the D5 receptor employs a signaling cascade other than AC/cyclic AMP in mediating at least some of its functions.
In light of the foregoing, there has been a long and gnawing problem of significant inconsistencies between D1-like agonist efficacies in stimulating cyclic AMP and the abilities of the drugs to induce electrophysiological responses or dopaminergic behaviours in various experimental models (Johansen et al., 1991; Arnt et al., 1992; Gnanalingham et al., 1995; Ruskin et al., 1998). Concerted efforts to clarify the endogenous coupling of the D5/D1B receptor, as well as continued characterisation of alternate D1-like signaling cascades are yielding new understanding of the pathways through which dopamine regulates brain function (see phosphoinositide signaling section below).
2.2.2. Cyclic AMP and protein kinase A stimulation
Cyclic-AMP-dependent protein kinase (PKA) is the immediate target of cyclic AMP in the series of events that constitute the AC/cyclic AMP cascade (see Figure 1). While it may be assumed that an increase in cyclic AMP automatically implies an increase in PKA activity, a direct demonstration of PKA activation provides a reliable confirmation of the continuity of the signaling cascade for a particular agonist or tissue. Dopamine and D1-like agonists increase PKA activity, and this effect is inhibited by SCH23390. While systematic pharmacological profiles have not been reported for a variety of D1-like agonists, existing results suggest that agonist efficacies in increasing PKA activity are generally consistent with actions on cyclic AMP accumulation.
PKA activity is important in the downstream regulation of various cellular processes. For example, using cyclic AMP mimics or direct activators or inhibitors of PKA, it has been possible to demonstrate a role for dopamine-sensitive PKA activation in the cellular regulation of sodium-dependent ion transporters (Aperia et al., 1987; Felder et al., 1990; Jose et al., 1995; Gomes and Soares-da-Silva, 2002), in the regulation of various ion channels (Drolet et al., 1997, Cantrell et al., 1999), in phosphorylation-dependent D1-like receptor regulation (Hausdorff et al., 1989), in cyclic AMP-response element binding protein (CREB) activation, and in amphetamine-mediated conditioned place preference response in rats (Beninger et al., 2003).
There are, however, instances where D1-like agonist responses correlated with cyclic AMP elevations but failed to correlate with activation of PKA. In some of these instances, an alternate cyclic AMP-dependent but PKA-independent pathway may be implicated, as further discussed below (see section on EPAC signaling).
2.2.3. Cyclic AMP and the DARPP-32/PP-1 system
Among its numerous substrates, a major physiological target of PKA phosphorylation is the dopamine and cyclic AMP-regulated phosphoprotein of 32 kDa (DARPP-32). In virtually all studied species, DARPP-32 is a cytosolic protein present in robust quantities within dopamine-responsive brain neurons, including practically all medium spiny neurons of the neostriatum and nucleus accumbens (Walaas et al., 1983; Ouimet et al., 1984; Ouimet and Greengard, 1990; Langley et al., 1997). While the striatum contains the highest density of DARPP-32 expression, the protein can be found at moderate levels throughout the neocortex, in the dentate gyrus of the hippocampus, and in the choroid plexus. There are also low levels of DARPP-32 in several other brain regions including hypothalamus and cerebellum. Several non-neuronal tissues known to express D1 receptors have also been shown to contain DARPP-32, including renal tubular epithelial cells, parathyroid hormone-producing cells of the parathyroid gland, and tanocytes (Hemmings, Jr. and Greengard, 1986; Matovcik et al., 1995).
Similar to the subcellular distribution of D1 receptors, DARPP-32 in the striatum is found in dendrites, axons and axon terminals, with very faint immunoreactivity demonstrated in some nuclei (Walaas and Greengard, 1984; Ouimet et al., 1984). Nevertheless, the regional and subregional distribution of this protein only partially matches the quantitative distribution of D1-like receptors, a fact that could limit the applicability of DARPP-32 as a universal mediator of D1-like physiology and pharmacology. Further, although DARPP-32 is expressed at high levels in both the striatonigral and striatopallidal neurons of the striatum, large cholinergic and medium-sized GABAergic interneurons are devoid of DARPP-32 immunoreactivity (Greengard et al., 1999). Seeing that the large cholinergic interneurons express the bulk of D1B/D5 receptors in the striatum, it may well be that DARPP-32 plays little if any role in mediating the actions of the D5/D1B subtype of D1-like receptors.
DARPP-32 is activated by PKA-mediated phosphorylation at its Thr34 site, which converts this phosphoprotein into a potent, high-affinity inhibitor of the multi-functional serine/threonine protein phosphatase, PP-1 (Greengard et al., 1999). Activation of D2-like receptors reduces the phosphorylated state of ‘nses were reversed by SCH23390, thus affirming their D1-like receptor dependence.
2.2.4. Cyclic AMP and EPAC signaling
Activation of PKA is the primary and generally expected consequence of D1-like receptor-mediated generation of cyclic AMP. However, an alternate cyclic AMP-dependent but non PKA-mediated pathway has been uncovered, and this involves the Exchange Protein Activated by Cyclic AMP (EPAC). EPAC is the GTP exchange factor for Rap1, a member of the Ras family of small GTP-binding proteins. Activation of EPAC promotes GTP binding to Rap1, thereby activating Rap1 for downstream signaling. Although manipulations that increase intracellular cyclic AMP can usually be expected to result in activation of both PKA and EPAC, it is possible to differentiate the actions of these proteins by employing membrane-permeable cyclic AMP analogs such as cpt-2-O-methyl-cyclic AMP [8-(4-chlorophenylthio)-2-O-methyl-cyclic AMP] which binds to EPAC but not PKA and therefore does not activate the latter (Helms et al., 2006).
A functional example implicating EPAC action is seen in D1-like receptor activation of epithelial sodium channels (ENaC) that regulate lung fluid clearance. As demonstrated by Helms and others (Helms et al., 2006), the PKA inhibitor H89 has little or no effect on dopamine-induced ENaC activation; conversely, the cyclic AMP analog, cpt-2-O-methyl-cyclic AMP, which activates EPAC but not PKA, mimics dopamine’s effects on ENaC activity. Thus, it is possible that EPAC and Rap1, which are usually associated with growth-factor receptors, are involved in an alternate cyclic AMP-mediated signaling pathway that increases ENaC activity in response to D1-like receptor stimulation.
2.3. Signaling via Phosphoinositide Pathways
2.3.1. Phosphoinositide signaling is a multipartite regulatory system
Receptor-coupled activation of phospholipase C (PLC) induces the hydrolysis of phosphatidylinositol-4,5-bisphosphate (PIP2) to generate the second messengers diacylglycerol (DG) and inositol-1,4,5-trisphosphate (IP3) (Berridge and Irvine, 1984; Berridge, 1984). Diacylglycerol serves as a stimulatory regulator of protein kinase C (PKC), while IP3 elevates cytosolic calcium by stimulating its release from intracellular storage sites such as the endoplasmic reticulum (Kishimoto et al., 1980; Berridge and Irvine, 1984; Berridge, 1984; Takai et al., 1984). While this represents the best known axis of phosphoinositide signaling, more recent studies depict a rather complex machinery comprising multiple points of regulation as well as downstream cascades emanating from the central PLC-linked axis. Essential components of these interconnected cascades are illustrated in Figure 2. Inositol 1,3,4,5-tetrakisphosphate which is formed by the further phosphorylation of IP3 regulates the metabolism of IP3 and works reciprocally with IP3 to restore cytosolic calcium back into intracellular storage sites (Joseph et al., 1987; Hill et al., 1988; Wilcox et al., 1993; Loomis-Husselbee et al., 1996). Inositol 1,2,3,4,5,6-hexakisphosphate, similarly formed from sequential phosphorylation of IP3, is also involved in calcium homeostasis and other actions through which it modulates cell differentiation (Shamsuddin, 1999). Deacylation of DG by release of the fatty acid at the C-2 position typically yields arachidonic acid which is the starting molecule in the synthesis of prostaglandins and other mediators. Conversely, removal of the C-1 acyl residue of phosphoinositide-derived diacylglycerol yields 2-arachidonoylglycerol, a full-efficacy endogenous agonist at CB1 endocannabinoid receptors. Phosphorylation of DG by DG kinase, on the other hand, yields phosphatidic acid; the latter can react with cellular cytidine triphosphate (CTP) to form cytidine diphosphate-diacylglycerol (CDP-DG). This reaction, catalyzed by CDP-DG synthase (CDS) generates the key intermediate in the synthesis of phosphatidylinositol and its various derived signaling lipids. In the synthesis of the phosphoinositides, CDP-diacylglycerol condenses with free myo-inositol (released from sequential dephosphorylation of IP3 or synthesized de novo from glucose-6-phosphate) to yield phosphatidylinositol. The latter is then sequentially phosphorylated to regenerate phosphatidylinositol-4-phosphate (PIP) and ultimately PIP2 which is the prime substrate for PLC.
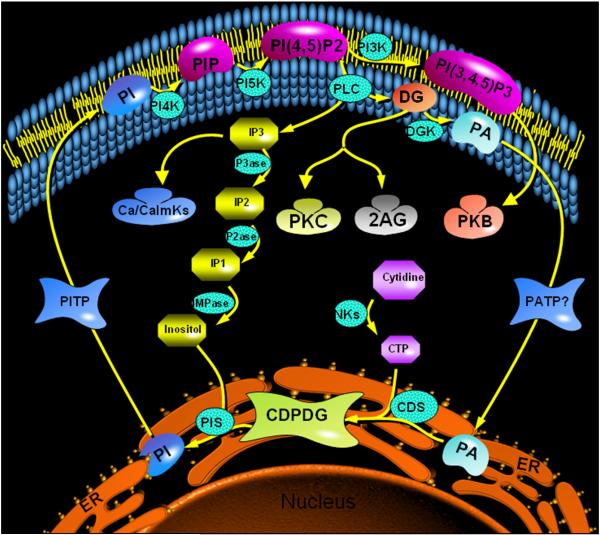
Phosphoinositide signaling cycle, showing the generation of second messengers and the resynthesis of phosphatidylinositide substrates. Synthesized in the endoplasmic reticulum (ER), phosphatidylinositol (PI) is transported by PI transport protein (PITP) to the cell membrane where it is phosphorylated to phosphatidylinositol-4-phosphate (PIP) and PI(4,5)P2 (phosphatidylinositol-4,5-bisphosphate) by respective kinases (PI4K and PI5K). A further phosphorylation of PI(4,5)P2 by PI-3-kinase yields PIP3 (phosphatidylinositol-3,4,5- trisphosphate) which functions to initiate downstream signaling via the Akt/PKB (protein kinase B) pathway. Phospholipase C (PLC)-mediated hydrolysis of PI(4,5)P2 generates the second messengers diacylglycerol (DG) and inositol trisphosphate (IP3). IP3 mobilizes calcium from the ER, resulting in activation of calcium-dependent calmodulin and downstream kinases (Ca/CamKs). DG activates protein kinase C (PKC) to initiate PKC-dependent downstream cascades, or DG could be converted to 2-arachidonoylglycerol (2AG), an endogenous ligand that initiates signaling via the CB1 endocannabinoid receptors. Sequential dephosphorylation of IP3 by respective phosphatases produces inositol bisphosphate (IP2), inositol monophosphate (IP1) and ultimately myo-inositol (inositol). DG not participating in immediate signaling is phosphorylated by DG kinase to produce phosphatidic acid (PA) which is then transported from the plasma membrane by unknown transport proteins to the ER. Here, the PA encounters cytidine triphosphate (CTP) formed by various nucleotide kinases from cytidine (or uridine) and, catalyzed by cytidine diphosphate diacylglycerol (CDPDG) synthase (CDS), PA and CTP react to yield CDP-DG. PI synthase (PIS) then catalyzes CDPDG reaction with myo-inositol to regenerate PI, thus completing the cycle. The PI cycle is by far the most prolific signaling system, generating at least four downstream cascades mediated via Ca/CalmKs, PKC, 2AG, and PKB. And in all, the rate-limiting step is the synthesis of CDPDG from CTP and PA.
The phosphoinositides implicated in PLC signaling are generally phosphorylated at the 1-, 4-, and 5-positions of the inositol moiety but not at the 3-position. However, an action of phosphatidylinositol-3-kinase (PI3K) on PIP2 produces phosphatidylinositol-1,3,4-trisphosphate (PIP3), an important signaling intermediate in the Akt/GSK pathways. Inositol phospholipids in the derivative forms of glycerophosphatidylinositides, also function to anchor various proteins to the cell membrane, thus determining their functionality. Hence, modulation of the activity of PLC, the critical step in phosphoinositide breakdown, or of CDS which is the rate-determining step in phosphoinositide synthesis, could have far-reaching consequences on cellular structure and signaling function.
2.3.2. Generation of Inositol phosphates and mobilization of intracellular calcium
Formation of Inositol Phosphates
Multiple laboratories have demonstrated that dopamine as well as D1-like receptor agonists can activate PLC-mediated phosphoinositide hydrolysis in native mammalian tissues (Felder et al., 1989a; Felder et al., 1989b; Dyck, 1990; Chen et al., 1992; Vyas et al., 1992a; Martin and Waszczak, 1993; Li et al., 1994; Kansra et al., 1995; Pacheco and Jope, 1997; Hussain and Lokhandwala, 1997; Friedman et al., 1997; Rosengarten and Friedhoff, 1998; Jin et al., 1998; Jope et al., 1998). These observations have been made in preparations of the rat kidney (Felder et al., 1989a; Felder et al., 1989b; Vyas et al., 1992a; Hussain and Lokhandwala, 1997), rat brain (Alexander and Crutcher, 1990; Undie and Friedman, 1990a; Undie and Friedman, 1990b; Arias-Montano et al., 1993; Martin and Waszczak, 1993; Li et al., 1994), mouse brain (Friedman et al., 1997; Undie, 1998), postmortem human brain (Wallace and Claro, 1993; Pacheco and Jope, 1997), fresh monkey brain (Panchalingam and Undie, 2001) and clonal cell lines of rat hippocampal origin (Jin et al., 1998). The findings are in contrast with some earlier reports that had implied a lack of association of dopamine with phosphoinositide hydrolysis in neural tissues or clonal cell lines (Pizzi et al., 1987; Cubitt et al., 1987; Pizzi et al., 1988; Rubinstein and Hitzemann, 1990). Some of the factors accounting for those early negative observations have been discussed in the literature (Undie and Friedman, 1990a; Undie and Friedman, 1990b; Undie and Friedman, 1992). Of further note are two reports in the 1980’s which suggested that dopamine and D2 agonists inhibited phosphoinositide hydrolysis in striatal slice preparations (Pizzi et al., 1987; Pizzi et al., 1988). We have been unable to reproduce those results in the absence of kynurenine, scopolamine, and glutathione – ingredients that were reported to be relevant for demonstrating an inhibitory effect of dopamine or quinpirole (Pizzi et al., 1988). These agents could have unpredictable effects on signaling events, as in the case of glutathione (Panchalingam and Undie, 2000; Undie et al., 2000). Hence, there is no clear evidence that D2 receptor stimulation, on its own, can inhibit basal or heterologous agonist-stimulated phosphoinositide hydrolysis.
Dopamine-sensitive phosphoinositide hydrolysis is associated with increased accumulation not only of IP3, but of IP2 and IP1 as well (Undie and Friedman, 1990b). While a significant increase in IP3 release is observed within two minutes of drug addition to prelabeled brain slice preparations, up to 16 minutes is required for the IP3 release to plateau. Conversely, IP1 accumulation begins to rise after several minutes and continues through approximately 60 min before achieving peak levels. Membrane assays involving the use of preformed PIP2 may show faster take-off times, but still require 30-60 min to reach peak levels of accumulation. These generally extended time courses of PLC activation are paralleled by the time course of agonist-induced GTPγS binding to activated Gq proteins (Panchalingam and Undie, 2001; Panchalingam and Undie, 2005; Mannoury la Cour et al., 2007). Hence, the temporal profiles may be a property of the Gq/PLC system rather than a limitation (or exaggeration) attributable to the coupled dopaminergic system. Indeed, even muscarinic and serotonergic stimulation of IP1 accumulation require similar time patterns for attainment of peak effects. Nevertheless, while muscarinic-stimulated IP3 accumulation reverts to baseline levels within four minutes of incubation with drug, dopaminergic stimulation of IP3 accumulation remains at measurable and significant levels well beyond 16 min of incubation with drug (Undie and Friedman, 1990b). Now, endogenous levels of PIP2 are typically depleted within a few minutes of agonist-mediated PLC stimulation. Hence a plausible explanation for the extended effects of the dopaminergic stimulus might be that dopamine agonists initiate a concomitant action that results in enhanced recycling or resynthesis of the phosphoinositide substrates (Undie, 1999).
The agonistic effect of dopamine on IP accumulation is dose-dependent and mimicked to various degrees of efficacy by apomorphine and SKF38393, but not by selective D2-like receptor agonists such as quinpirole (Undie and Friedman, 1990a; Undie and Friedman, 1990b). The effects of dopamine and SKF38393 are mediated through a D1-like dopaminergic mechanism inasmuch as the responses are inhibited by SCH23390 but not by antagonists selective for D2-like dopaminergic, 5HT2 serotonergic, α-noradrenergic, or muscarinic acetylcholine receptors (Undie and Friedman, 1990a; Undie and Friedman, 1990b; Undie and Friedman, 1992). The foregoing receptors represent the predominant monoaminergic receptor systems that are coupled to phosphoinositide hydrolysis and/or with which dopamine or SKF38393 could interact within the tissues that were used in these experiments.
Brain distribution studies indicate regionally dependent variations in agonist efficacies in the rodent brain, with significant effects in the striatum, frontal cortex, hippocampus, and especially high levels of coupling in the amygdala – a region of the brain that lacks significant dopamine-sensitive AC activity (Mailman et al., 1986; Kilts et al., 1988; Undie and Friedman, 1990b). The pattern of regional distribution of the PLC response only partially account for [3H]SCH23390 or [3H]SKF38393 binding sites in specific brain regions such as the striatum, implying that the PLC response may be mediated by only a subset of total SCH23390-sensitive binding sites (Undie, 1998).
Dopamine agonist stimulation of phosphoinositide hydrolysis does not result from dopamine-mediated AC activation inasmuch as the response is not mimicked by cyclic AMP analogs or activators of AC or PKA (Undie and Friedman, 1994). In experiments where some twenty dopamine agonists were tested for parallel effects on inositol phosphate accumulation and cyclic AMP formation in striatal preparations, the drugs were shown to exhibit significant differences in efficacy, ranging from compounds such as SKF81427, SKF38393 and fenoldopam which exhibit as much efficacy as DA, to other compounds such as SKF85174, SKF86284, and SKF83822 which lack significant effects on inositol phosphate formation (Undie et al., 1994). These differential second messenger effects have been corroborated at the level of specific G protein activation (Wang et al., 1995; Jin et al., 2001; Panchalingam and Undie, 2005; Mannoury la Cour et al., 2007).
Following stimulation with dopamine or D1-like agonists, brain tissues show increased activation of various G proteins. Dopamine itself stimulates Gs, Gi, and Gq; a D2-like receptor agonist such as quinpirole stimulates only Gi; whereas D1-like receptor agonists stimulate Gs and Gq (Wang et al., 1995; Jin et al., 2001). Notably, SKF83959, a D1-like receptor agonist devoid of stimulatory actions on AC, is able to significantly stimulate Gq – the G protein that is associated with stimulation of PLC – thus further indicating that an ability to stimulate AC is not required for D1-like agonist stimulation of Gq/PLC signaling (Matsumoto et al., 1998; Zaworski et al., 1999; Panchalingam and Undie, 2001). The G protein or phosphoinositide effects of D1-like agonists have been observed in clonal cell lines, in brain tissues tested in vitro, and in rat brain tissues in vivo (Wang et al., 1995; Jin et al., 2001). Moreover, the effects have been demonstrated not only in rodents, but also in monkey and human brain tissues (Pacheco and Jope, 1997; Panchalingam and Undie, 2001). Thus, D1-like agents that stimulate inositol phosphate accumulation probably do so by stimulating a D1-like receptor that couples through Gq to activate PLC-mediated phosphoinositide hydrolysis.
Intracellular calcium mobilization
Intracellular calcium plays a critical role in the regulation of virtually every aspect of cellular function, and transmembrane calcium channels constitute the principal means by which calcium ions gain entry into cells. However, cytosolic calcium may also be mobilized from intracellular stores through the action of IP3. D1-like agonists can modulate cellular calcium function through multiple mechanisms that include IP3-mediated mobilization of intracellular calcium stores, and protein kinase-mediated phosphorylation of channel proteins. As reported by Ming and colleagues (Ming et al., 2006), the selective PLC-effective D1-like agonist, SKF83959, dose-dependently evokes a sustained augmentation of basal intracellular calcium concentrations ([Ca2+]i) in hippocampal primary neuronal cultures. This effect is strongly attenuated by the PLCβ inhibitor U73122, but not by the inactive analogue U73343. The effect is also blocked by the specific D1-like receptor antagonist SKF83566, but not by antagonists of dopamine D2-like, serotonin 5HT1C/5HT2, α-adrenergic, or muscarinic cholinergic receptors. The fast phase of D1-like agonist-induced calcium mobilization is blocked by thapsigargin-induced depletion of intracellular calcium, but is only partially reduced by exclusion of extracellular calcium from the assay medium. Conversely, the late phase of the agonist-induced [Ca2+]i response is considerably reduced by cadmium (Cd2+) and nifedipine which are voltage-gated calcium channel antagonists, but not by tetrodotoxin, a Na+ channel blocker. These effects are typical of a calcium mobilizing mechanism that involves agonist-induced PLCβ-mediated phosphoinositide hydrolysis to release IP3 that then causes intracellular Ca2+ release. More recently, a similar dopamine-mediated IP3/ Ca2+/calmodulin-dependent protein kinase cascade has been associated with enhanced synthesis of fibroblast growth factor and neuroprotective effects in cultured astrocytes (Zhang et al., 2009).
The ability of various isoforms of the IP3 receptor to mobilize intracellular calcium is intricately subject to influences from diverse factors, not the least of which is the phosphorylation of the Ca2+ channel by different kinases. For instance, PKA-dependent phosphorylation of IP3R1 leads to enhanced sensitivity of the receptor/channel to IP3 activation (Nakade et al., 1994; Wojcikiewicz and Luo, 1998; Wagner et al., 2003; Tang et al., 2003). Further, calcium channels of the P/Q type are reportedly selectively inhibited by agonist activation of D1-like receptors; this effect attenuates glutamate release in immature rat cholinergic basal forebrain neurons (Momiyama and Fukazawa, 2007), but augments GABA release in rat striatum probably via cyclic AMP/PKA-dependent phosphorylation of the channels (Arias-Montano et al., 2007).
Direct D1-like receptor effects on calcium channels is evident in dopamine agonist-induced activation of increased calcium currents of the L-type calcium channel and increased Ca2+ uptake in rat striatal neurons (Surmeier et al., 1995), medium spiny neostriatal neurons (Hernandez-Lopez et al., 1997), D1 receptor-transfected GH4C1 cells (Liu et al., 1992; Surmeier et al., 1995), D5 receptor-expressing subthalamic neurons (Baufreton et al., 2003), rat frontal cortex neurons (Young and Yang, 2004), and primary renal proximal tubule cells (Han et al., 2007). D1-like agonists can convert L-type Ca2+ inhibitors into Ca2+ influx facilitators in cultured striatal neurons (Eaton et al., 2004). Some of these responses can be mimicked by introduction of cyclic AMP analogs (Liu et al., 1992; Surmeier et al., 1995) and blocked by inhibitors of PKA (Surmeier et al., 1995), suggesting that phosphorylation of calcium channels probably contributes to such responses. D1-like agonist-associated phosphorylation appears to exert varying effects on different species of calcium channels. For instance, neuronal P and Q channels may be enhanced (Gross et al., 1990; Mogul et al., 1993) or inhibited (Surmeier et al., 1995) by phosphorylation. These differential outcomes may be due to inherent differences in P/Q channels in various neuronal tissues or concomitant phosphorylation of other regulatory proteins (Bargas et al., 1994; Surmeier et al., 1995).
2.3.3. Production of Diacylglycerol and Activation of Protein Kinase C
Diacylyglycerol is an additional second messenger produced through PLC-mediated phosphoinositide hydrolysis (see Figure 2). Unlike IP3 which diffuses through the cytosol to stimulate calcium release from the endoplasmic reticulum, the more hydrophobic diacylglycerol largely remains associated with the plasma membrane where it participates in activating calcium-dependent protein kinase (PKC). PKC activation requires intracellular calcium and is enhanced in the presence of certain phospholipids such as phosphatidylserine. Hence, the diacylglycerol arm of the PLC/phosphoinositide cycle works in tandem with the IP3 arm in that cytosolic calcium mobilized through the action of IP3 may be deployed not only for the regulation of calmodulin and calcineurin cascades but also for the activation of PKC signaling.
Few studies outside of the author’s laboratory have examined the diacylglycerol arm of phosphoinositide signaling either in response to dopamine or as part of other receptor systems. A 1999 study that examined several parameters of CDP-diacylglycerol (CDP-DG) accumulation as an index of agonist-stimulated diacylglycerol formation demonstrated that dopamine and SKF38393 significantly and dose-dependently stimulate CDP-DG accumulation (Undie, 1999). These effects are inhibited by the D1-like receptor antagonist SCH23390. SKF38393-induced CDP-DG response is significantly reduced by neomycin and reversed by myo-inositol, implying that most if not all of the product is generated from the phosphoinositides. Consistent with this notion, the effects of SKF38393 on CDP-DG are significantly abrogated in the presence of diacylglycerol kinase inhibitors that prevent the reutilization of PLC-generated diacylglycerol. The latter observation should also preclude any notion of an exclusive role for phospholipase-D or de novo phosphatidate synthesis in the dopaminergic response, seeing those sources are less likely to be affected by inhibition of diacylglycerol kinase.
CDP-diacylglycerol probably plays multiple signaling roles besides serving as a reservoir for released diacylglycerol or as a metabolic substrate for the resynthesis of the phosphoinositides. Most cells are intolerant to diacylglycerol accumulation. Released diacylglycerol that is not converted to CDP-DG, therefore, is deacylated, albeit to products that may mediate signaling functions in their own right. The action of phospholipase A2 removes the arachidonoyl moiety at the 2-position to release free arachidonic acid, a signaling molecule that is also a crucial physiological intermediate in the biosynthesis of eicosanoids such as the prostaglandins (Shimizu and Wolfe, 1990; Khan et al., 1995; Lo et al., 1996). Removal of the 1-acyl function from diacylglycerol produces the endocannabinoid 2-arachidonoylglycerol which is a potent, full-efficacy cannabinoid receptor agonist with diverse functional implications (Sugiura et al., 1995; Giuffrida et al., 1999; Di, V et al., 2000; Stella and Piomelli, 2001; Alger, 2005; Sugiura et al., 2006). Interestingly, an increase in cellular 2-arachidonoylglycerol levels is a normal concomitant of activated phosphoinositide hydrolysis reactions (Stella and Piomelli, 2001; Fride, 2002; Solinas et al., 2008). Thus, through these variously interdependent actions, the IP3 and diacylglycerol arms of the PLC/phosphoinositide signaling system could implement cooperative, complementary, or even synergistic programs in the cell (de Chaffoy et al., 1984). Indeed, the possibility that phospholipid signaling may contribute to the synergistic interactions among dopamine D1-like and D2-like receptors has been suggested by us and others (Undie, 2002; Kirchheimer et al., 2007), and continues to receive vigorous attention in this laboratory.
Protein kinase C is the physiological target of diacylglycerol released through the phosphodiesteratic cleavage of PIP2 (Nishizuka, 1988; Walaas and Greengard, 1991; Dekker and Parker, 1994). A requirement or concomitant of PKC activation is the translocation of the enzyme from the cytosol to the cell membrane compartment. Thus, positive PKC stimuli induce translocation and/or enhance the phosphorylating activity of the enzyme. Dopamine D1-like receptor stimulation has been associated with increased translocation and activation of protein kinase C in rat striatal or prefrontal cortical neurons (Simpson and Morris, 1995; Young and Yang, 2004), in opposum kidney cells (Gomes and Soares-da-Silva, 2002; Pedrosa et al., 2004; Gomes and Soares-da-Silva, 2004), and in renal epithelial cells or proximal tubule (Kansra et al., 1995; Yao et al., 1998; Nowicki et al., 2000). In a study where D1-like receptor agonists were reported to inhibit PKC activity as assayed in cytosolic fractions, it was also noted that the agents concomitantly increased PKC activity in the particulate or membrane fraction (Giambalvo and Wagner, 1994). As it became clearer in subsequent studies, the apparent reduced activity in the cytosol was probably due to enzyme activation and translocation to the membrane fraction, rather than agonist-mediated inhibition of enzyme activity. Hence, the opposite but more appropriate inference, that dopamine activates PKC, has gained acceptance. Dopamine-induced PKC activation probably serves a physiological role inasmuch as blockade of PKC activation interferes with several dopaminergic effects, including regulation of Na+/K+-ATPase (Shahedi et al., 1992; Vyas et al., 1992b), suppression of L-type Ca2+ channel spikes through PKC-mediated phosphorylation and consequent inactivation of the channel (Obejero-Paz et al., 1998; McHugh et al., 2000), and modulation of NR1a/NR2B glutamate receptor function in conjunction with PKA and PSD-95 (Gu et al., 2007).
2.3.4. Resynthesis of phosphatidylinositides and enhancement of Akt cascades
Dopaminergic stimulation of phosphoinositide metabolism produces conventional increases in inositol phosphate and diacylglycerol second messengers; however, there is also concomitant and concentration-dependent increase in the synthesis or resynthesis of inositol phospholipids (Undie, 1999). Thus the ratio of newly labelled phosphoinositides compared with inositol phosphates is higher following dopamine receptor stimulation than for other phosphoinositide-linked monoamine receptors, including alpha norepinephrine, 5HT2 serotonin, and muscarinic acetylcholine receptors. The picture emerging is one where, following dopamine receptor stimulation, there is increased phospholipase C-mediated breakdown of PIP2 to yield IP3 and diacylglycerol, the latter is then recycled via phosphatidic acid and CDP-DG for the resynthesis of phosphatidylinositol. If dopamine is able to mobilize CDP-DG from additional sources (a proposition that has not been tested), then such may contribute to the disproportionately higher accumulation of CDP-DG relative to IP3 in dopamine-exposed tissues. The model further envisions that the synthesized phosphatidylinositol is readily available to the actions of the various phosphoinositide kinases, hence its subsequent conversion to PIP, PIP2 as well as PIP3 through the actions of their respective kinases. Thus, the ultimate fate of the dopamine-enhanced phosphatidylinositol synthesis depends on the relative activities of the PLC-coupled versus PI3K coupled pathways. Consequently, dopamine could prime both the PLC and PI3K systems for enhanced responsiveness by increasing the supply or replenishment of substrates used for the synthesis of signaling molecules that are critical to each of these cascades.
Direct or indirect modulation of the provision of substrates for the phosphatidylinositol-3-kinases could have extensive and profound effects on functions regulated via PIP3-modulated cascades, including cellular differentiation, survival, growth, proliferation, transformation, anti-apoptotic machinery, generation of super-oxides, migration, and adhesion (Toker et al., 1994; Domin and Waterfield, 1997; Toker and Cantley, 1997; Vanhaesebroeck et al., 2001), as well as vesicular trafficking and targeting of proteins to specific intracellular compartments (De Camilli et al., 1996). There is limited evidence that dopamine directly enhances the enzymatic activity of PI3K (Waly et al., 2004). However, dopamine has been shown to enhance Akt activity and to regulate Akt’s downstream targets. Akt is a member of the serine threonine kinases that is regulated through direct phosphorylation by PI3K or through direct stimulation by PIP3 (Scheid and Woodgett, 2001). Akt possesses a specific protein domain known as the Pleckstrin homology domain to which PI3K-generated PIP3 binds, thereby facilitating translocation of the Akt molecule from the cytosol to the plasma membrane where it is activated by phosphorylation at two critical Thr308 and Ser473 residues. Detaching from the membrane, the activated Akt targets specific cytosolic substrates such as glycogen synthase kinase-3 (GSK3) and mTOR, or further translocates into the nucleus where it phosphorylates other substrates including a number of transcription factors (Cross et al., 1995; Frame and Cohen, 2001; Brunet et al., 2001; Manning and Cantley, 2003; Sui et al., 2008). Both GSK-3β-mediated and mTOR-mediated cascades are critical cellular pathways involved in regulating a wide range of functions, including cellular metabolism, protein synthesis, apoptosis or cellular pro-survival, transcription factor activation, cell cycle regulation, and control of synaptic strength (Welsh et al., 1996; Pap and Cooper, 1998; Manning and Cantley, 2003; Bhat et al., 2004; Kim and Kimmel, 2006; Beurel and Jope, 2006; Jope et al., 2007; Sui et al., 2008).
Akt is implicated in dopamine signaling, and D1-like receptor stimulation inhibits GSK-3β activity probably via activation of Akt (Yu et al., 2008). Loss of D1-like receptor-mediated inhibitory action on GSK-3β is thought to underlie the neuronal morphological changes observed in rabbits prenataly exposed to cocaine (Zhen et al., 2001). Consistent with this inference, the D1-like receptor agonist SKF38393 was shown to inhibit GSK-3β activity in frontal cortical neurons of control but not test rabbits exposed to cocaine in utero (Gil et al., 2003).
Akt has also been associated with D1-like receptor signaling via mechanisms apparently not linked to PI3K. In cultured primary neurons of the striatum, SKF38393 induced a swift activation and nuclear translocation of Akt; this was accompanied with rapidly elevated phosphorylation of Akt on the Thr308 residue that is associated with its kinase effects (Brami-Cherrier et al., 2002). Further, unlike the activation induced by growth factors, this D1-like agonist-mediated activation of Akt was not associated with enhanced PI3K activity inasmuch as wortmannin, an inhibitor of PI3K, had no effect on the D1 agonist action. While it is possible that some PI3K-independent actions of D1-like receptors may be mediated through MAP kinase pathways (Zhen et al., 1998; Nomura et al., 2001; Zanassi et al., 2001), there is need for additional studies to delineate the relative contributions of the various signaling cascades that are initiated by D1-like agonists.
3. BIOPHYSICAL MODELS OF D1-LIKE RECEPTOR SIGNALING
Evidently, dopamine D1-like receptors can couple to multiple signaling systems which, at the minimum, comprise adenylyl cyclase-regulated and phosphoinositide-mediated cascades. What then might be the biophysical mechanisms (and associated physicochemical features) that enable the D1-like receptors to couple to multiple signaling cascades and retain the ability to function coordinately upon dopamine release, especially in tissues where the receptors are coexpressed? Based on our observations and synthesis of the literature, we have identified four models that could explain with various degrees of effectiveness or experimental support, the observed effects of D1-like agonists on multiple signaling pathways. These models are discussed in relation to classical G-protein-mediated metabotropic signaling via AC and PLC. While D1-like agonists modulate ionic conductances, it appears that these ionic effects are downstream of an initial action on metabotropic effectors (Undie, 2000), hence the ionic effects are not discussed in the present models.
3.1. Distinct Coupling Mechanisms for D1 and D5 Receptors
The first model is the rather conventional assumption that the D1 and D5 receptors employ a classical G-protein-transduced membrane machinery to couple to specific signaling pathways which are separately activated – implying that one pathway does not have to be active in order for the other to be activated. Either the same receptor molecule may select to couple to AC or PLC depending on its biological circumstance, or one receptor subtype couples to one and only one signaling cascade (AC or PLC) under physiologic conditions (but, like other G protein-coupled receptors, may become promiscuous under certain non-physiologic conditions such as when expressed in artificial cell lines or with an abundance of alternate G proteins). To facilitate discussion, we refer to these two variants as “distinct and selectable” (each subtype can choose AC or PLC as needed) and “distinct and separable” (one receptor subtype uses AC while the other uses PLC), respectively.
Notwithstanding their present pharmacological similarities, D1 and D5 receptors differ in several respects, as highlighted above. Depending on how physiologically substantive these differences turn out to be, they could provide the basis for a signaling model in which the two receptor subtypes distinctly couple through specific G proteins to regulate separate signaling cascades. In other words, it is possible that D1 and D5 receptors respectively couple to AC and PLC in physiological tissues. The “distinct and separable” signaling model, illustrated in Figure 3 for D5, D2, and D1 receptor subtypes, is supported by a growing body of evidence.
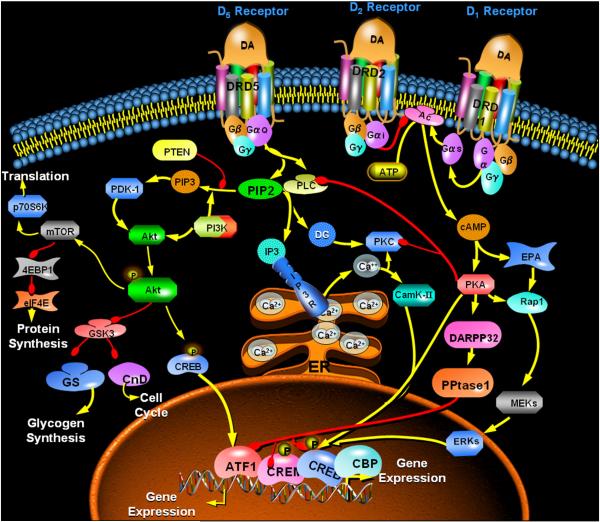
Schematic of dopamine-sensitive signaling via adenylyl cyclase (AC), phospholipase C (PLC), and phosphatidylinositol-3-kinase (PI3K) systems. This simplified view of the signaling pathways shows key downstream mediators and the various opportunities for interaction or integration among the pathways. Note the inhibitory effect of cyclic AMP-dependent protein kinase (PKA) on the activity of PLC, an action that provides a mechanism for crosstalk between the AC system and the PI/PLC systems. Besides hampering inositol trisphosphate (IP3)- mediated intracellular calcium mobilization (which would inhibit DARPP32 Thr34 phosphorylation via calmodulin/protein phosphatase 2B), it may also be possible that inhibition of PIP2 (phosphatidylinositol-4,5-bisphosphate) utilization via PLC could shunt the phospholipid to the PI3K pathway. Red arrows depict inhibition, while yellow arrows depict stimulation. Abbreviations: DA, dopamine; DRD1, Dopamine receptor D1 subtype; DRD2, Dopamine receptor D2 subtype; DRD5, Dopamine receptor D5 subtype; cAMP, cyclic AMP; PKA, protein kinase A; EPAC, Exchange protein activated by cyclic AMP; PPtase1, protein phosphatase 1, MEKs/ERKs, mitogen-activated kinases; ATF1/CREM/CREB/CDP, illustrative transcription-regulatory factors; CamK-II, calcium-calmodulin-dependent protein kinase type II; DG, diacylglycerol; IP3R, inositol trisphosphate receptor; Ca2+, calcium ions within and exiting the endoplasmic storage; PIP3, phosphatidylinositol-3,4,5-trisphosphate; PTEN, phosphatase and tensin homolog; PDK-1, phosphoinositide-dependent kinase-1; Akt, protein kinase B; GSK3, glycogen synthase kinase-3; CcnD, cyclin D; mTOR, mammalian target of rapamycin; 4EBP1/eIF4E/p70S6K, downstream mediators to protein synthesis.
In physiologic tissues, the D1 receptor has been consistently associated with AC signaling but not PLC signaling. Experimental manipulations that deplete or inactivate D1 receptors result in the loss of dopamine-sensitive AC signaling but not dopamine-sensitive PLC signaling, suggesting that the latter response is not mediated via the D1 receptor (Friedman et al., 1997; Undie et al., 2000). Further, dopamine and some D1-like receptor agonists stimulate both the AC-coupled Gs and the PLC-coupled Gq proteins, but the receptor subtypes that mediate these responses appear to be separate. In a series of exquisite experiments by Friedman and colleagues (Wang et al., 1995; Friedman and Wang, 1996), it was demonstrated that dopamine and D1-like agonists stimulated [35S]GTPγS binding to the AC-coupled Gs and the PLC-coupled Gq proteins in rat striatal and frontal cortical tissues, but only Gαq was stimulated in the hippocampus or amygdala. When solubilized tissue membranes from the cortex or hippocampus were subjected to immunoprecipitation with anti-Gs or anti-Gq antisera followed by receptor binding analyses, SCH23390 binding sites were detected in both anti-Gs and anti-Gq-induced immunoprecipitates from the cortex, and in the anti-Gq precipitate from the hippocampus; however, only the anti-Gs-mediated immunoprecipitates also showed immunoreactivity for the D1A receptor. This implies that the D1-like receptor that is associated with the anti-Gq-induced immunoprecipitates is not the D1A subtype. In these same experiments, it was noted that incubations with quinpirole resulted in the activation of Gi, but neither Gs nor Gq, hence arguing against the presence in this preparation of a D1/D2 oligomer that can stimulate Gq/PLC in response to either D1-selective or D2-selective agonists. These observations provide convincing evidence that the D1A receptor mediates dopamine and D1-like agonist stimulation of Gs/AC signaling but not the stimulation of dopamine-sensitive PLC signaling.
Current evidence for D5 receptor coupling to AC signaling in native brain tissues is neither conclusive nor does it exclude the possibility of coupling to other signaling cascades. Indeed, following D1A receptor knockout, there are extensive reductions (but not a complete loss) in D1-like binding sites and loss of D1-like agonist stimulation of AC (Mailman et al., 1986; Friedman et al., 1997; Montague et al., 2001). This may imply that the D5 receptor contributes little to brain dopaminergic function or, more likely, that the D5 receptor constitutes a smaller fraction of total SCH23390 binding sites in some brain regions such as the striatum, and that this site couples to a signaling cascade other than AC. With regard to relative receptor density, the fact that D5 receptors are expressed mostly in neuronal perikarya and are localized intracellularly implies that a substantial portion of the receptor presence may not be detected in ordinary autoradiographic studies. Thus, the observation by Montague and colleagues (Montague et al., 2001) of near-total loss of [3H]SCH23390 autoradiography in D1A knockout animals probably underestimates the true contribution of the D5 subtype to brain D1-like receptor populations and function. With regard to AC coupling, it is noted that following EEDQ-induced inactivation of SCH23390 binding sites in adult rats, whence there is as much as 75% loss of D1 binding sites and complete loss of AC stimulation in response to D1 agonists, there is no loss of D1-like agonist stimulation of phosphoinositide hydrolysis (Rosengarten and Friedhoff, 1998; Undie et al., 2000). Hence, only a portion of D1-like binding sites in the striatum may be required for mediating the full D1-like agonist response on PLC in this tissue. Such would be consistent with the existence of functional spare receptors in D1 systems (Battaglia et al., 1986; Meller et al., 1988; Watts et al., 1995). Alternatively, the observations may suggest the existence of different D1-like receptor subtypes one of which is more susceptible to EEDQ and couples to AC while the other is less sensitive to EEDQ and couples to PLC. Were these two components of D1-like receptors D1 and D5, respectively, then the ability of the D5 receptor to promiscuously couple to multiple G proteins may explain its mediation of D1-like agonist-stimulated cyclic AMP formation in some transfected cell lines (Sidhu et al., 1998b; Sidhu and Niznik, 2000).
The possibility that the D5 receptor may be the subtype that mediates dopamine-sensitive phosphoinositide signaling was recently highlighted by observations in D5 receptor knockout mice. Specifically, dopamine or D1-like agonists failed to significantly induce IP accumulation or CDP-DG production in D5-null mice compared to wild-type controls (Sahu et al., 2009), even though D5 knockout animals have shown substantial D1-like agonist stimulation of AC. Moreover, in experiments where intracellular imaging of agonist-induced phosphoinositide hydrolysis was assessed in organotypic striatal cultures, D1-like agonist-induced CDP-DG accumulation was confined to the cell soma and proximal axons of the neurons (Undieh et al, Unpublished observations), which is consistent with the perikaryal subcellular distribution of the D5 receptor but not the D1 receptors that are typically localized to dendrites (Weiner et al., 1991; Yung et al., 1995). A D5-PLC link has also been repeatedly implicated in studies of Gq activation or Ca2+ mobilization that permit separate molecular manipulations or observations of the D1 and D5 subtypes (Zheng et al., 2003; Baufreton et al., 2003; So et al., 2009).
The inference that the D5 receptor may be the dopamine D1-like receptor subtype that physiologically couples to PLC is consistent with the known characteristics of dopamine-induced phosphoinositide signaling and D5 receptor expression among the brain regions. For instance, both receptor expression and agonist-induced signaling are relatively higher in the hippocampus than in the striatum, with intermediate effects in the prefrontal cortex (Undie and Friedman, 1990b). The D5 receptor can couple to Gq-like G proteins in various cell lines or in renal brush border membranes (Sidhu and Niznik, 2000), the receptor directly modulates calcium currents and burst firing in the subthalamic nucleus (Baufreton et al., 2003), and it frequently exists in extrasynaptic microdomains associated with neuronal inositol-1,4,5-trisphosphate-sensitive calcium stores (Paspalas and Goldman-Rakic, 2004). The D5 inference is also supported by previous experiments involving the expression of a D1-like receptor encoded by striatal mRNA in Xenopus oocytes. Mahan and coworkers (Mahan et al., 1990) found that injection of rat striatal mRNA into Xenopus oocytes led to the expression of a D1-like receptor coupled to inositol phosphate production and Ca2+ mobilization. However, expression of the cloned rat D1 receptor in the oocytes led to the production of cyclic AMP, but not Ca2+ mobilization, suggesting that the D1 receptor was not linked to the phosphoinositide response (Monsma, Jr. et al., 1990). Moreover, using size fractionation techniques, it was shown that mRNA encoding the striatal phosphoinositide-linked D1-like receptor was between 2.5-3.0 kb in size, thus distinguishing it from the 4.1 kb mRNA fragment that encodes the rat D1 receptor. Interestingly, the rat D5 receptor is encoded by an mRNA that is ~3 kb in size (Tiberi et al., 1991), in close agreement with the size of the mRNA encoding the phosphoinositide-linked D1-like receptor identified using the oocyte expression system. Hence, the Ca2+ response observed in those early oocyte expression experiments probably involved the D5 receptor subtype.
A separate coupling of D1 and D5 receptors to distinct signaling cascades is more in tune with numerous distinct functional observations such as: (i) the opposite effects of these receptors on LTP v. LTD (Centonze et al., 2003a), (ii) differential intrastriatal distribution of the receptors among chemically and functionally different neuronal phenotypes (Le Moine et al., 1991; Kawaguchi et al., 1995; Rivera et al., 2002; Centonze et al., 2003b), (iii) differential interactions with other receptors and neuromodulators (White et al., 1999; Liu et al., 2000), (iv) effects of antisense oligonucleotide-induced downregulation on signaling responses in adult animals (Undie, 1998), (v) regulatory actions on hypothalamic function (Apostolakis et al., 1996a), (vi) modulation of lordosis following specific antisense oligonucleotide treatments and local application of agonists in the brain (Apostolakis et al., 1996b), (vi) effects on motor control (Sibley, 1999; O’Sullivan et al., 2004); and (vii) significantly different phenotypes resulting from genomic inactivation of the receptors (Waddington et al., 1995; Nicola et al., 1996; Waddington et al., 2001; Holmes et al., 2001).
The foregoing inferences present a new opportunity to interpret or reinterpret the role of the D5 receptor as the D1-like receptor subtype that mediates a subset of dopamine’s effects. Such effects that were previously held suspect might include the regulation of peripheral blood pressure (Hollon et al., 2002), enhanced acetylcholine release in the hippocampus (Hersi et al., 2000), stimulation of pituitary prolactin secretion (Saller and Salama, 1986; Fabbrini et al., 1988; Schoors et al., 1991), and modulation of mucosal vulnerability to psychosomatic ulcerogenic insults (Hunyady et al., 2001). At the behavior level, congenic D5 receptor mutant mice show marked reductions in grooming, a characteristic D1-like dopaminergic response that is nevertheless not cyclase-mediated (O’Sullivan et al., 2005). Moreover, orofacial movement topographies inducible in naïve animals by SKF83959 (which does not stimulate AC), are severely disrupted in congenic D5 mutant mice (Tomiyama et al., 2006). Nevertheless, such mice continue to demonstrate grooming and episodic seizure activity in response to the AC-effective D1-like agonist, SKF83822. Hence, the loss of D5 signaling correlates more to behavioral mediation via PLC than via AC, thus providing further support to the notion that the D5 receptor regulates a defined subset of physiological dopaminergic responses, as was previously thought for the dopamine-linked phosphoinositide signaling response (Deveney and Waddington, 1995; Adachi et al., 1999; Clifford et al., 1999; Undie et al., 2000; Makihara et al., 2007).
In sum, then, the evidence favours the distinct and separable coupling of D1/D1A receptors to AC signaling and of the D5/D1B receptor subtype to PLC signaling in native brain (and possibly renal) tissues.
3.2. Activity-Dependent Selectivity of Signaling Pathways
This model seeks to interpret observations that have revealed significantly different responsiveness between the dopamine-sensitive AC and PLC cascades. In general, a primary messenger may activate multiple and distinct transmembrane signaling pathways to achieve diverse functional outcomes within a given tissue, provided that the signaling pathways are sufficiently differentiable in sensitivity and responsivity. In this context, sensitivity is the inverse of the ligand concentration (or stimulation frequency) necessary to elicit a biologically or statistically significant (or 50% of maximal) response; while responsivity refers to the efficiency with which receptor activation is translated into a physiological response – mathematically inferred from the maxima of the concentration (or stimulation frequency)-response curve. The concentration of ligand at which either system attains maximal response may differ between the systems; however, the magnitudes of the response maxima for the two signaling systems are irrelevant to the operation of the model (seeing, for example, the unlikelihood that any biological system would need to routinely function at maximum levels). Thus, sensitivity and responsivity could be as much a property of the signaling system as potency and efficacy are properties of a pharmacological ligand. Physiologically, this sort of model would be applicable where one signaling system is deployed to regulate function at tonic to moderate levels of neuronal activity and the second system is mobilized to supplement or supplant the first system during occasions of high neuronal stimulation.
For the dopamine system, the activity-dependent selectivity model implies that D1-like receptor signaling through AC and/or PLC pathways is determined by the intensity and duration of synaptic dopamine activity. Thus, at tonic to moderate levels of dopamine neuron stimulation (or agonist concentration), one pathway is active, whereas at higher levels or longer durations of stimulation the second pathway is mobilized to supplement or supplant the base system. While it is not necessary to the operation of this model that each signaling pathway be activated through a different subtype of D1-like receptors, such is not precluded either. However, the receptor subtypes, conformations, or states that couple to the different pathways must be differentiable in terms of sensitivity and/or responsivity. Indeed, from a design perspective, using separate receptor subtypes might provide for more optimal regulation of the system, for example, by deploying differential density of expression, cellular and subcellular distribution, or sensitivity to modulation by co-transmitters. Several experimental observations lend credence to the possible operation of this model within the dopamine system.
Pharmacological studies of AC and PLC signaling reveal concentration-dependent effects of dopamine and selective D1-like agonists at each signaling response (Undie et al., 1994). Agonist potencies between the two pathways, however, can differ by as much as an order of magnitude (Undie et al., 1994). Generally, the AC system shows the greater sensitivity. Some of the sensitivity difference may be accounted for by the experimental use of whole cells or tissue slices for PLC assays versus membrane preparations for AC assays, and by the availability of good “traps” (i.e., phosphodiesterase inhibitors) for cyclic AMP versus poorly effective “dams” (LiCl) for inositol phosphate or diacylglycerol analytes. Nevertheless, when both the AC and PLC assays were conducted in brain slice preparations, the AC response still saturated at lower dopamine concentrations than the PLC response, although the PLC response showed the greater fold increase above control (Undie and Friedman, 1994; Wang et al., 1995; Panchalingam and Undie, 2005). Additionally, AC stimulation appears to attain maximal response within minutes, whereas PLC stimulation, measured as accumulation of either inositol phosphates or CDP-diacylglycerol, continues to show increases up to an hour or longer (Undie and Friedman, 1990b; Undie and Friedman, 1994; Undie, 1999). Thus, not only must sensitivity and responsivity (or potency and efficacy) be considered, we must also integrate a temporal factor in the mix of parameters that may functionally differentiate the two D1-like receptors or dopamine-sensitive signaling pathways.
Temporal studies of agonist-stimulated GTP binding, an assay that indicates effective receptor stimulation provide support for the activation-driven selectivity model. D1-like agonist stimulation of Gs, the AC-coupled G protein, occurs relatively fast and reaches peak levels of activation within 15 min (Wang et al., 1995). Conversely, D1-like agonist stimulation of the PLC-coupled Gq occurs with a lag time of 10-15 min, and attains peak responses only after 60 min (Wang et al., 1995; Panchalingam and Undie, 2005). Nevertheless, the eventual maximal percentage stimulation of Gq is several fold greater than the maximal percentage stimulation of Gs (Panchalingam and Undie, 2001; Panchalingam and Undie, 2005). Table 1 shows summary data indicating that assaying Gq protein activation at longer time points can “increase” the measurable response from 0-20% above control seen by 15 minutes to 400-700% above control as seen after 120 min. The integration of comparable agonist concentrations over a longer duration of activation is apparently more favorable to Gq/PLC coupling in contrast with the coupling to Gs/AC. This brings to the forefront a question about the role of time – duration – in functional responsivity. Is time important only to allow for adequate diffusion of drug to the site of action, or may signaling events cumulate with time so as to attain a threshold or extend a response? For instance, Gq-mediated PLC stimulation usually consists of a fast and transient phase followed by a slower but prolonged phase. While this is easily explicable on the basis of diffusion-related impediments in the case of whole cells or organ systems, the fact that these temporal patterns can occur even in cell-free preparations implies that they may be a property of the signaling apparatus rather than the result of physicochemical impediments to drug diffusion (Thorne and Nicholson, 2006; Thorne et al., 2008). Such a phenomenon of cumulative signaling should warrant further exploration. But, for the present discourse, it is intriguing that dopamine could achieve a level of functional sequencing by employing two receptor subtypes that signal through a sensitive, fast-onset, short-duration cascade such as the AC system and a comparably less sensitive, slower-onset and longer-duration system such as the PLC system.
Table 1
Agonist potencies and efficacies in stimulation of [35S]
G Protein Stimulation (GTPγS Binding) | ||||||||||||
---|---|---|---|---|---|---|---|---|---|---|---|---|
Gs-Favored (15-min) Assay | Gq-Favored (120-min) Assay | |||||||||||
Potency (μM) | Efficacy (%) | Potency (μM) | Efficacy (%) | |||||||||
Compound | Str | FCx | Hip | Str | Fcx | Hip | Str | FCx | Hip | Str | FCx | Hip |
Dopamine | 7 | 26 | 46 | 54 | 48 | 47 | 44 | 41.2 | 40.7 | 512 | 528 | 787 |
SKF38393 | 22 | 2 | 11 | 13 | 6 | 5 | 45 | 22.3 | 39.2 | 452 | 476 | 693 |
SKF83959 | ND | ND | ND | 0 | 2 | 9 | 4.5 | 1.1 | 1.5 | 79 | 76 | 101 |
Fenoldopam | 0.8 | ND | ND | 19 | 4 | 2 | 8.9 | 2.1 | 3.2 | 81 | 80 | 104 |
Abbreviations: Str, Striatum; FCx, Frontal cortex; Hip, Hippocampus; ND, Not Determinable. Adapted from Panchalingam and Undie (Panchalingam and Undie, 2005)
Another line of support comes from a 1995 study showing that elevation of intracellular cyclic AMP inhibits subsequent D1-like agonist stimulation of phosphoinositide hydrolysis (Undie and Friedman, 1994). To obtain the same levels of inositol phosphate stimulation, agonist concentrations had to be raised. Cyclic AMP-induced inhibition of PLC signaling is evident when cellular cyclic AMP levels are increased by incubations with cell-permeable cyclic AMP analogs, by in situ activation of AC with forskolin, or by treatment with Gs-effective dopaminergic agonists that stimulate cyclic AMP (Undie and Friedman, 1994). As such, blockade of cyclic AMP action with RpcAMPS can release the system from cyclic AMP-mediated inhibition, allowing Gq-effective D1-like agonists to induce significantly greater activation of PLC. Co-stimulation of phosphoinositide hydrolysis, however, does not appear to modulate subsequent cyclic AMP formation. Taken together, these findings suggest that the cyclase system may be attuned to function at tonic to moderate levels of dopaminergic activity, whereas colocalized Gq-mediated activity may not be evident until moderate to high levels of dopaminergic stimulation are attained. Such higher levels of synaptic dopamine may be achieved endogenously during high phasic neuronal stimulation or following administration of agents like psychostimulants that inhibit dopamine clearance resulting in markedly elevated synaptic levels of the transmitter.
A series of neurochemical and functional studies from different laboratories indicate that the response profiles obtained by the activation of D1-like receptors can vary qualitatively depending on the amount of agonist administered (Cai and Arnsten, 1997; Zahrt et al., 1997; Arnsten, 1997; Granon et al., 2000; Lidow et al., 2003). Rather than induce quantitatively commensurate responses until a peak is attained, varying the agonist concentration past a critical point could rather elicit divergent or opposite effects on a given functional endpoint. Calabrese and colleagues have applied the term hormesis to the phenomenon in which a substance ordinarily evoking stimulatory responses at low doses is found to generate inhibitory responses at high doses or vice versa (Calabrese and Baldwin, 2002). Hormesis exists in two general forms of dose response relationships: the monotonic dose response which involves responses that generally proceed from zero or above and move on unidirectionally, and the bitonic dose response which presents a bi-directional or biphasic form where the dose response curve assumes either a U, inverted U, or J-shape, perhaps depending on the included dose segments of measurement (Calabrese, 2002). Stimulation of D1-like receptors in monkeys and rats executing working memory tasks associated with the prefrontal cortex has been shown to produce an inverted U-shaped dose-response curve (Lidow et al., 2003). In this instance, either suboptimal stimulation (Sawaguchi and Goldman-Rakic, 1991; Seamans et al., 1998; Kozlov et al., 2001) or over-activation (Zahrt et al., 1997; Arnsten and Goldman-Rakic, 1998) would tend to interfere with task execution. Other studies in monkeys have shown similar inverted U responses to D1-like receptor activation by delay-related activity of prefrontal cortical neurons which are strengthened at low but attenuated at high doses (Sawaguchi and Goldman-Rakic, 1994; Williams and Goldman-Rakic, 1995). A notable observation about hormesis in D1-like receptor function is that it occurs with D1-like receptor stimulation under relatively normal physiology. Thus, an ability to mobilize an alternate signaling cascade that supplants the base system under excessive levels of activation could serve as a self-managed circuit-breaker of sorts. Indeed, it has been speculated that this phenomenon may underlie some of the ordinary differences observed in higher cognitive abilities (Vijayraghavan et al., 2007). While the neurophysiological or molecular explanations for these phenomena are yet to emerge, constructs such as the present activity-dependent selectivity hypothesis could help to systematize future investigations to help link molecular mechanisms with functional outcomes.
3.3. Dynamic Compartmentalization of Signaling Components
The existence of multiple membrane folds and compartments within the cell creates extended surfaces or pockets for inserting various molecules whose juxtaposition or segregation might be crucial for normal cell function. Although most GPCRs including D1-like receptors are generally seen as being localized on the plasma membrane, at basal cellular conditions the majority of these receptors occur mainly in intracellular compartments, as visualized in kidney and heart cells (O’Connell et al., 1995; Ozono et al., 1996; Brismar et al., 2002; Kruse et al., 2003), and in native brain tissues (Bloch et al., 1999). This raises the possibility that, in a cell expressing multiple receptor subtypes, subpopulations of the same receptor subtype or members of separate receptor subtypes could be localized to different subcellular compartments. From here the receptors may traffic to the cell membrane as needed, or while within the intracellular compartment the receptors could participate in local intracellular signaling events that could be equally significant to normal cell function (Bloch et al., 1999; Sadowski et al., 2008). In rat cerebrocortical tissues, D1 and D5 receptors appear to differentially distribute to distinct subcellular compartments, apparently facilitated and abated by the presence of lipid rafts and reductive thiol functions (Voulalas and Undieh, Unpublished observations). Interestingly, dopamine or a D1-like receptor agonist could induce inter-compartmental redistribution of the D1 but not the D5 (or even the D2) receptor subtype. This may imply that there are functional consequences emanating from the subcellular localization of dopamine receptor subtypes.
Lipid rafts appear to be critical for maintaining the intracompartmental distribution or segregation of receptors and other signaling elements and membrane proteins. The primary constituents of these membranous microdomains are structural lipids, notably sphingolipids, cholesterol, and glycolipids; in addition there are specific proteins whose major distinguishing features are their insolubility in nonionic detergents at 4 °C as well as their light buoyancy on sucrose gradients (Little and Teyler, 1998; Ming et al., 2006). Lipid rafts are thought to provide an enabling environment for the formation of functional complexes among various molecular components of signal transduction cascades (Lisanti et al., 1994; Simons and Ikonen, 1997; Shaul and Anderson, 1998; Ostrom et al., 2000; Galbiati et al., 2001; Anderson and Jacobson, 2002). It has been possible to isolate from raft-like structures various signaling molecules that are known to be involved in D1-like receptor cascades, including heterotrimeric G-proteins, GTPases, GRK, AC, PLC, PKC and PKA (Sargiacomo et al., 1993; Smart et al., 1995; Song et al., 1996; Carman and Benovic, 1998; Bathori et al., 1999; Razani et al., 1999; Carman et al., 1999; Ostrom et al., 2000). While these mediators are not necessarily restricted to dopaminergic systems, their presence in these cellular substructures further attests to the significance of membrane molecular segregation or packaging in cellular signaling function.
Among the numerous varieties of lipid rafts that have been identified, the best known are the caveolae, which are polymerization products of caveolin and cholesterol occurring primarily on invaginations of cellular surfaces (Lisanti et al., 1994; Shaul and Anderson, 1998; Ostrom et al., 2000; Galbiati et al., 2001; Anderson and Jacobson, 2002). Caveolae facilitate the creation of subcellular compartments for various signaling constituents such as adaptors, scaffolds and enzymes; such compartmentalization enhances the competency and efficiency of receptor coupling to multiple effector systems (Lisanti et al., 1994). In this regard, Trivedi and colleagues (Trivedi et al., 2004) showed that functionally competent D1A receptors with the conserved ability to couple with G-proteins as well as stimulate cyclic AMP accumulation and inhibit Na/K-ATPase in rat proximal tubules are conscripted to caveolar plasma membranes rich in Na/K-ATPase by the agonist action of dopamine. The dopamine agonist action appears to be mediated through D1-like receptor-cyclic AMP signaling pathways. In other experiments, Yu and coworkers (Yu et al., 2004) uncovered a link between caveolin-2β and human D1-like receptors whereby stimulation with fenoldopam, a D1-like receptor agonist, led to an increase in the amounts of caveolin-2β that were associated with the receptors. Agonist-induced D1 receptor-mediated AC activation was observed at much higher degrees in lipid rafts as opposed to plasma membranes not associated with lipid rafts. Moreover, following antisense-mediated knockdown of caveolin-2 expression, the agonist action of fenoldopam on cyclic AMP production was significantly reduced. Based on these studies, it appears that the primary location of human D1 receptors, at least in these cells where they were exogenously expressed, is in lipid rafts and that heterologous as well as endogenous expression of these receptors might be closely linked to and modulated by caveolin-2. In contrast, the D5 receptors are predominantly intracellularly localized and their behaviour in response to agonist stimulation remains to be fully elucidated.
Besides their differential steady-state localization, D1-like receptors traffic between the cell membrane and other cellular compartments, and these movements are relevant to ultimate receptor function. D1-like receptors as other GPCRs, are synthesized cytoplasmically and then are transported to the plasma membrane of cell bodies and dendrites (Levey et al., 1993; Hersch et al., 1994; Liu and Lasater, 1994; Caille et al., 1996). Within minutes following acute activation by dopamine, D1-like receptor localization is further modified by translocation of the receptors from neuronal surfaces to cytoplasmic endosomal compartments with consequent reduction in the density of membrane receptors (Mantyh et al., 1995a; Mantyh et al., 1995b; Sternini et al., 1996; Dumartin et al., 1998; Bernard et al., 1998; Bloch et al., 1999). Subsequently, the internalized receptors are degraded or are resensitized by being recycled back into the plasma membrane. The complex process of intracellular trafficking contributes to the regulation of the abundance of D1-like receptors at the cell surface where typically receptor-ligand interactions occur. Of additional interest is the possibility that trafficking of D1 and D5 receptors, or of AC-coupled and PLC-coupled sites, may differ, with attendant functional implications. For example, Kruse and colleagues (Kruse et al., 2003) showed that dopamine-induced D1-like receptor translocation from intracellular compartments to the plasma membrane is associated with altered activation of protein kinase C. This finding is consistent with our own recent observation that nocodazole and other microtubule inhibitors block dopamine or D1 agonist stimulation of PLC signaling in brain tissue probably by preventing cytoskeletal transport of mediators to the cell membrane (Undieh et al, Unpublished observations). While a clear understanding of these observations must await additional mechanistic studies, the observations are nevertheless consistent with the view that diverse signaling mediators are segregated among subcellular compartments, and that the regulated movement of the molecules among the compartments may be a natural and usual concomitant of receptor activation.
For receptors that could interact with multiple signaling partners, the subcellular localization of the receptor could become an important determinant of the receptor’s signaling response in certain cells or under particular conditions. As an example, following expression in intracellular compartments of COS-7 cells, the D1 receptor constitutively heteromerizes with the NR1 subunit of the NMDA receptor, whereas the D5 receptor does not undergo any such interaction (Fiorentini et al., 2003). This difference, which is attributed to differences in the C-terminal structure of the two receptors, enables the D1 receptor to be primarily targeted to the plasma membrane, to co-exist with the NMDA receptor in the postsynaptic density, and to resist agonist-induced cytoplasmic sequestration (Fiorentini et al., 2003). On the other hand, N-glycosylation is critical for functional cell surface expression of the D5 receptor in HEK-293 cells, whereas inhibition of N-glycosylation has no effect on trafficking of the D1 receptor to the plasma membrane (Karpa et al., 1999). As earlier highlighted, these kinds of differences between the D1 and D5 receptors are probably not accidental, but rather appear to be consistent with (or even deterministic of) the differing functional roles of the receptors. Future studies to further clarify these potential distinctions should incorporate experimental designs that deliberately compare or contrast D1 versus D5 receptor subtypes as well as AC-mediated versus PLC-related mediators and end points. For now, it remains speculative that signaling differences could result from relative differences in receptor subtype expression in a given tissue or cell, trafficking to the cell membrane, subdistribution into lipid rafts or other membrane compartments, localization within or near synaptic clefts, functional selectivity for a given test ligand (Ryman-Rasmussen et al., 2007), association with any receptor activity-modifying proteins, propensity for cross-phosphorylation, readiness to undergo intracellular sequestration, and susceptibility to endosomal degradation or functional recycling to active sites on the cell membrane.
3.4. Receptor Oligomerization for Recruitment of Alternate Signaling Cascades
Structural and biochemical evidence points to the innate capacity of diverse GPCRs to endogenously form molecular complexes consisting of two subunits (dimers) or multiple subunits (oligomers). The subunits may structurally represent the same receptor entity (hence, homomers) or the units may come from two distinct receptors belonging to the same or different families (heteromers) (Gouldson et al., 1998; Hebert et al., 1998; Bouvier, 2001). Dopamine D1 receptors can form homodimers, heterodimers, and possibly oligomers as well (Seeman et al., 1992; George et al., 1998; Lee et al., 2000; Liu et al., 2000; O’Dowd et al., 2005; Kong et al., 2006). Interestingly, if our observation of subcellular segregation of D1 and D5 receptors in neocortical neurons can be generalized to other tissues, then it is unlikely that these two species would be able to come close enough to form a D1-like heterodimer under normal circumstances in such tissues. Nevertheless, D1-like receptors, especially the D1 receptor, enjoy a rich repertoire of heteromerization with D2-like dopaminergic, A1 adenosine, and other receptor species (Franco et al., 2000; Lee et al., 2004; So et al., 2005; Rashid et al., 2007a; Juhasz et al., 2008).
While homodimerization of D1-like receptors could enhance functional cooperativity particularly involving receptor stimulation and signal transduction amplification (Kong et al., 2006), heteromerization is thought to be motivated by a need to generate functional complexes with agonist affinity and signaling mechanisms separate from those of the original individual receptor subunits (George et al., 2002; Kong et al., 2006). Nevertheless, signal amplification may not be the exclusive rationale for homomerization, hence both homomers and heteromers may modulate receptor trafficking (Nelson et al., 2001; Uberti et al., 2003; Hague et al., 2004; Kong et al., 2006), and intracellular localization (O’Dowd et al., 2005), as well as serve a signal-enhancing function by increasing the number of receptor units that can be available for activation by a single ligand or agonist molecule.
The functional consequences of D1 receptor heteromerization with D2 receptors has been explored by George and colleagues (Lee et al., 2004; So et al., 2005; Rashid et al., 2007b). Notably, D1/D2 oligomers are detectable under unstimulated conditions, and demonstrate patterns of cell surface localization, internalization, and transactivation that are distinct from the properties of homooligomeric D1 or D2 receptors (So et al., 2005). Functionally, the D1/D2 heteromeric complex responds additively to selective D1 and D2 agonists, and elicits a novel Gq/phospholipase C/Ca2+ signal that is not seen with either receptor separately expressed and stimulated by its cognate agonist (Lee et al., 2004; Rashid et al., 2007b). It has recently been demonstrated that the D5 receptor also can oligomerize with the D2 receptor (So et al., 2009). However, in contrast with D1/D2 oligomerization where agonist-induced calcium mobilization is facilitated, D5/D2 heteromerization results in inhibition of D5-mediated calcium mobilization. This is consistent with our recent demonstration that the D5 receptor natively couples to Gq/PLC signaling (Sahu et al., 2009) and that a knockout of the D2 receptor does not alter D1-like agonist stimulation of phosphoinositide hydrolysis (Undieh et al; Unpublished observations). Thus, it is demonstrated once again that D1 and D5 receptors may show close similarities with regard to ligand selectivity, but the structural differences at the C terminal tails of these two D1-like receptors exert significant influences on the molecular interactions and signaling outputs of the receptors.
While several models have been proposed to explain the general intermolecular interactions that lead to receptor oligomerization (Bouvier, 2001), it is noteworthy that for the D5 receptor a C-terminal segment appears to be the portion that engages in interactions with the GABAA receptor. Hence, the full length receptor may not be required in order for oligomeric interactions to occur. Also, George and colleagues (George et al., 1998) demonstrate that a peptide homologous only to the t6 transmembrane domain of the D1X receptor inhibited D1 receptor function. Hence, one wonders if similar regulation might exist for the D5 receptor, and if this might hint at a possible biological significance for the existence of D5 receptor pseudogenes. Two of these pseudogenes which code for non-functional abbreviated forms of the D5 receptor have been detected and characterized to be about 98 % identical to each other and 95 % identical to the D1 receptor (Weinshank et al., 1991; Grandy et al., 1991; Grandy et al., 1992). Might physiological or pathological expression of the receptor pseudogenes serve a role in modulating authentic D5 receptor expression, intracellular compartmentalization, trafficking to the plasma membrane, affinity for dopamine ligands, or coupling to specific signal transduction cascades?
The thought then is that even if the known D1-like receptors individually coupled to similar signaling cascades, their divergent oligomerization with distinct populations of heterologous receptors could produce sufficiently differentiated signaling outcomes to justify their co-expression (and hence simultaneous stimulation by dopamine) in various tissues.
3.5. Systems Integration for Exquisite Functional Regulation
Whether D1-like receptors couple indiscriminately to multiple transducers, or they couple distinctively to separate signaling systems, the receptor subtypes and associated pathways must perform in a coordinate fashion in order to maintain functional integrity of the target organs. This is specifically critical for cells or tissues where the receptors are co-expressed. Both the AC and PLC systems in general include intracellular cascades whose mediators are known to undergo extensive regulatory crosstalk. A full discussion of the associated mechanisms is beyond the scope of the present review, but such mechanisms may include cross-phosphorylation, genomic transactivation, and induced translocation of the signaling mediators. Thus, the fact that D1 and D5 receptors may induce multiple distinct signaling pathways does not necessarily imply that the systems are functionally isolated. By mobilizing separate streams of mediators and providing for points of integration among them, the multiple receptor/transducer/effector systems provide for exquisite homeostatic regulation of dopaminergic function under basal physiological conditions or in attempts to deal with the vicissitudes of the external environment.
Mechanistically, while multiple models of signal coupling have been discussed, it is not impossible that several of these may operate simultaneously. Such would then provide another level of integration and control among the systems. For example, the receptors may couple to distinct signaling cascades, but the sensitivity and time-cumulative responsivity of the systems may be such as to also permit functional sequencing as an activity-dependent mode of regulation. Another example is where signaling components may be held within lipid rafts or other cellular compartments for normal signaling, but can be redistributed to other subcellular compartments to avoid excessive activation or degradation. While these are speculative gestures, one hopes that such would motivate more outside-the-box thinking that could lead to innovative experimental designs and findings.
As commonly known in dopamine neurobiology, it is rare that D1-like dopamine receptors function in isolation from their D2-like siblings. This is demonstrated consistently in behavioral responses to co-administration of selective agonists or selective antagonists. What has further confounded the field for decades is the fact that at the biochemical level D1-like and D2-like receptors induce opposing effects on cyclic AMP, whereas at the behavioral level most responses are synergistically induced by combined administration of subclass-selective agonists. How D1-like and D2-like receptors and functions may be integrated at the molecular, biochemical, or behavioral levels is another question that must be considered. Based on existing literature and our own results, we have envisioned a working model of interactive dopamine receptor signaling that is helping to focus our interpretations of various experimental observations. This integrative signaling model encompasses the effects of dopamine D1-like and D2-like agonists in both AC-mediated and PLC-related signaling pathways. An illustration of the model is shown in Figure 4. By the model, the D1-like receptors that stimulate AC are designated as D1AC, the D1-like receptors that stimulate PLC or otherwise couple to phosphoinositide metabolism as D1PL, the D2 receptors that inhibit AC as D2AC, while D2NC represents those D2 receptors that enhance various neurolipid-dependent signaling events such as those induced by the diacylglycerol-derived endocannabinoid, 2-arachidonoylglycerol. These designations are functional, with no structural identity or distinction implied or required between either the D2 categories or the D1 categories. For a given functional output, such as vacuous perioral movement (VPM) behavior, which is synergistically induced by D1/D2 agonists, administration of a broad-acting D2 agonist, such as quinpirole, would activate both the AC-coupled (D2AC) and the neurolipid-mobilizing (D2NC) D2-like receptors. Activation of D2AC leads to inhibition of a D1 agonist-induced AC stimulation by the AC-coupled D1 receptors (D1AC). Given that AC activity normally maintains a tonic inhibition of PLC activation, at least in the striatum as earlier discussed (Undie and Friedman, 1994), the inhibition of D1AC by D2AC should lead to disinhibition of the tonic AC effects on D1PL. A D1PL-active agonist such as SKF83959 would thus act unopposed by AC to elicit the functional output. By reducing AC-mediated inhibition of PLC, and possibly by enhancing neurolipid-mediated signaling, the D2 agonist produces a synergistic or at least additive functional effect with a D1PL agonist (Piomelli et al., 1991). Thus, a D2AC agonist (with or without concomitant D2NC activation) could produce synergism or additivity when co-administered with a D1PL (or nonselective D1AC/D1PL) agonist. Oppositional D2/D1 effects may still be exerted on those functional responses that are directly mediated by D2AC or by D1AC, consistent with the oppositional biochemical effects of these receptor subtypes on AC activation.
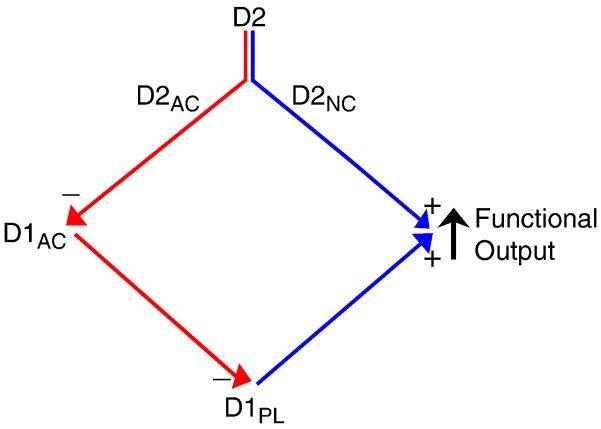
Schema of integrative dopamine receptor signaling. The schema depicts a model of how the separate and sometimes oppositional biochemical effects of D1-like and D2-like receptors could lead to cooperative mediation of function by invocation of the phosphoinositide-linked dopamine system. Stimulation of cyclase-linked D2-like receptors (D2AC) inhibits stimulation of cyclase-linked D1-like receptors (D1AC) which in turn inhibit stimulation of phosphoinositide-linked D1-like receptors (D1PL). Thus coadministration of a D2AC agonist and a D1PL agonist could yield additive or synergistic effects whether in the presence or absence of D1AC stimulation. Moreover, there are D2-like receptors not linked to cyclase inhibition (D2NC), that may function by ion channel modulation or via coupling to signaling neurolipids such as the endocannabinoid system. These receptors afford further opportunities for interaction with the D1PL system. For example D1PL stimulation generates diacylglycerol which is the endogenous precursor for the synthesis of 2-arachidonoylglycerol, a full-efficacy ligand at CB1 endocannabinoid receptors, and this system is responsive to D2-like receptor stimulation. Here again, D2-like (D2NC) and D1PL agonism could produce cooperative functional output without the need for concomitant D1AC stimulation.
The presented model relates to the biochemical mechanisms that may underlie the functional interactions of dopamine receptor subtypes within the same cell or tissue. When examining function at the behavioral level, it is not always clear whether we are looking at a single brain nucleus or whether we are seeing the net effects of actions from multisynaptic circuits. Thus, there are bound to be significant limitations with the model. Evidently, we are for now seeing only dimly. As new information emerges from additional studies and the evidence begins to weigh in one direction or the other, we should be able to expand and refine the model to better approximate the reality.
4. Summary and Prospects
4.1. What we think we know
Positive evidence accumulated over the last decade is enabling us to begin to emerge from the debate on whether or not D1-like receptors were of any physiological significance. This emergence, however, is being guarded by the concomitant observations that D1-like receptors consist of more than one species, and that D1-like receptors elicit more than one distinct signaling response. Regarding the receptor subtypes, the findings in toto would suggest that D1/D1A and D5/D1B receptors exist and function as paternal twins with substantial similarities, but probably not as maternal clones that have equivalent structure, distribution, effector coupling, or physiological function. Notable among the coupling systems are the classical coupling through Gs to the activation of adenylylcyclase, and the more recent uncovering of a link to the stimulation of Gq and PLC. Further down from each of these proximal mechanisms, however, there are additional diversifications.
In the AC system, there is evidence that not all actions initiated by AC stimulation and cyclic AMP formation feed through PKA or DARPP-32 systems, but may involve EPAC signaling. Most D1-like agonists, with the exception of SKF83959, stimulate AC signaling, but this should not be a surprise since this action contributed to the definition or selection of the agents as D1 agonists. Notably, however, agonist efficacies in stimulating cyclic AMP accumulation do not consistently correlate with cellular or behavioral effects of the drugs, implying that multiple actions generally contribute to the functional output following D1-like agonist application.
There is evidence for D1-like agonist activation of MAPK signaling and for modulation of various ion channels and ion exchange mechanisms. These effects appear to generally result from downstream actions of the primary metabotropic signaling products.
At PLC-related pathways, the actions of dopamine or D1-like agonists are associated with the accumulation of inositol phosphate and diacylglycerol messengers. In addition, there is agonist-related induction of CDP-diacylglycerol and phosphatidylinositide synthesis beyond the extent that could arise solely from recycling of PLC-mediated hydrolysis products. While agents such as fenoldopam and SKF81427 are fully efficacious in this system, other D1 agonists including SKF85174 and SKF86822 that display high agonistic efficacy in the AC system are devoid of significant effects in the phosphoinositide system. Currently, SKF83959 is the most potent and selective agonist at the phosphoinositide system, although the drug’s efficacy is lower relative to dopamine or the classic D1 agonist, SKF38393.
There are plausible leads regarding the nature of the coupling between each D1-like receptor subtype and downstream signaling responses. Endogenous cyclic AMP responses to D1-like agonist stimulation in the rodent brain can be accounted for by the presence and action of the D1/1A receptor subtype, but not necessarily the D5/1B subtype. On the other hand, dopamine-sensitive phosphoinositide responses relate more to the D5 receptor inasmuch as D5-knockout but not D1-knockout tissues show significant reductions in the ability of D1-like agonists to induce phosphoinositide-related responses (Friedman et al., 1997; Undie, 1998; Sahu et al., 2009). Further, reliable evidence linking the D5 receptor to AC stimulation in native physiological tissue is yet to emerge, given that D1 agonists lose the ability to stimulate AC in D1-knockout animals (even though the D5 receptors should still be present). Physiologically, therefore, it appears that D1 but not D5 receptors are crucial for dopaminergic coupling to AC, while D5 but not D1 receptors are crucial for dopaminergic coupling to PLC.
Several previously demonstrated dopaminergic responses appear to be associated with D5 and/or PLC signaling. Notable among these are the involvement of dopamine in regulation of peripheral blood pressure, enhanced acetylcholine release in the hippocampus, stimulation of pituitary prolactin secretion, and certain orofacial movement topographies that are inducible in naïve animals by SKF83959.
We have proposed an integrated signaling model in an attempt to capture and organize the multifaceted findings emanating from D1-like (and almost inextricably D2-like) dopaminergic signaling. Altogether, the evidence suggests that the AC and PLC pathways of dopaminergic signaling are mediated through different receptor subtypes, crosstalk with each other, and subserve different subsets or programs among dopamine-regulated physiological functions.
4.2. What we wish we knew
Notwithstanding the significant progress achieved in understanding dopamine’s actions and functions, especially in the brain, profound and crucial questions remain to be addressed. Perhaps the most vexing of the pending questions relates to the nature of the molecular entity that mediates the stimulatory effects of dopamine D1-like agonists on phosphoinositide hydrolysis. Is it the D5, and only the D5, receptor? Or is it a receptor complex such as an oligomer formed homologously or with some other receptor or cellular protein? Is the coupling specific to Gq/PLC, or does it promiscuously couple to other effector systems and, if so, in what tissues and under what conditions?
The ancillary observation of significant dopaminergic effects on CDP-diacylglycerol open a new line of inquiry regarding the underlying mechanisms and functional implications of the response. Considering its pivotal role in relation to diverse metabolic and signaling pathways, CDP-diacylglycerol signaling will ultimately be found to be crucial to various dopamine-regulated physiological functions. A clarification of the signaling relationship between dopamine and CDP-diacylglycerol could shed some new light on even some dopamine-associated disorders such as addiction, depression, and Parkinson disease.
It would definitely be helpful if we could have the pharmacological tools to acutely and selectively manipulate each of the D1-like receptors. While the creation of receptor knockout or knockdown mutant animals has helped to some extent in delineating D1 from D5 effects, possible adaptive changes during development of the mutant animals sometimes complicate the interpretation of the subsequent experimental data. There is need for agonists and antagonists that would sufficiently discriminate between D1 and D5 receptors both in vitro and in vivo. Progress in this regard has been painfully slow, but could improve through increased support for exploratory synthesis of novel compounds.
D1 and D5 receptors (as well as D1-like and D2-like receptors) are often co-expressed, especially among various brain tissues. While several models have been proposed here on how the coexpressed receptors may function, given their divergent coupling to signaling cascades, these models need to be tested. In particular, it should be of significant interest if it were possible to validate the proposition that D1 and D5 receptors (or AC and PLC cascades) may be differentially regulated by the intensity and duration of dopaminergic activation.
Lastly, we wish there were, and we knew, a defined spectrum of dopaminergic functions and dysfunctions that may be attributed to each of the D1-like receptors and to the dopamine-sensitive adenylylcyclase and phosphoinositide signaling pathways. Such understanding is crucial for a full appreciation of the neuropathological and pharmacotherapeutic potential of D1 and D5 receptor targets.
Footnotes
Publisher's Disclaimer: This is a PDF file of an unedited manuscript that has been accepted for publication. As a service to our customers we are providing this early version of the manuscript. The manuscript will undergo copyediting, typesetting, and review of the resulting proof before it is published in its final citable form. Please note that during the production process errors may be discovered which could affect the content, and all legal disclaimers that apply to the journal pertain.
5. References
- Adachi K, Ikeda H, Hasegawa M, Nakamura S, Waddington JL, Koshikawa N. SK&F 83959 and non-cyclase-coupled dopamine D1-like receptors in jaw movements via dopamine D1-like/D2-like receptor synergism. Eur J Pharmacol. 1999;367:143–149. [Abstract] [Google Scholar]
- Agui T, Chase TN, Kebabian JW. Identification of D1-dopamine receptor in chicken embryo retina with [125I]SCH 23982. Brain Res. 1988;452:49–56. [Abstract] [Google Scholar]
- Alexander GE, Crutcher MD. Functional architecture of basal ganglia circuits: neural substrates of parallel processing. TINS. 1990;13:266–271. [Abstract] [Google Scholar]
- Alger BE. Endocannabinoid identification in the brain: studies of breakdown lead to breakthrough, and there may be NO hope. Sci STKE. 2005;2005:e51. [Abstract] [Google Scholar]
- Andersen PH, Jansen JA. Dopamine receptor agonists: selectivity and dopamine D1 receptor efficacy. Eur J Pharmacol. 1990;188:335–347. [Abstract] [Google Scholar]
- Andersen PH, Nielsen EB, Scheel-Kruger J, Jansen JA, Hohlweg R. Thienopyridine derivatives identified as the first selective, full efficacy, dopamine D1 receptor agonists. Eur J Pharmacol. 1987;137:291–292. [Abstract] [Google Scholar]
- Anderson RG, Jacobson K. A role for lipid shells in targeting proteins to caveolae, rafts, and other lipid domains. Science. 2002;296:1821–1825. [Abstract] [Google Scholar]
- Andringa G, Drukarch B, Leysen JE, Cools AR, Stoof JC. The alleged dopamine D1 receptor agonist SKF 83959 is a dopamine D1 receptor antagonist in primate cells and interacts with other receptors. Eur J Pharmacol. 1999;364:33–41. [Abstract] [Google Scholar]
- Aperia A, Bertorello A, Seri I. Dopamine causes inhibition of Na+-K+-ATPase activity in rat proximal convoluted tubule segments. Am J Physiol. 1987;252:F39–F45. [Abstract] [Google Scholar]
- Aperia A, Hokfelt T, Meister B, Bertorello A, Fryckstedt J, Holtback U, Seri I. The significance of L-amino acid decarboxylase and DARPP-32 in the kidney. Am J Hypertens. 1990;3:11S–13S. [Abstract] [Google Scholar]
- Apostolakis EM, Garai J, Clark JH, O’Malley BW. In vivo regulation of central nervous system progesterone receptors: cocaine induces steroid-dependent behavior through dopamine transporter modulation of D5 receptors in rats. Mol Endocrinol. 1996a;10:1595–1604. [Abstract] [Google Scholar]
- Apostolakis EM, Garai J, Fox C, Smith CL, Watson SJ, Clark JH, O’Malley BW. Dopaminergic regulation of progesterone receptors: brain D5 dopamine receptors mediate induction of lordosis by D1-like agonists in rats. J Neurosci. 1996b;16:4823–4834. [Europe PMC free article] [Abstract] [Google Scholar]
- Ariano MA, Sibley DR. Dopamine receptor distribution in the rat CNS: elucidation using anti-peptide antisera directed against D1A and D3 subtypes. Brain Res. 1994;649:95–110. [Abstract] [Google Scholar]
- Arias-Montano JA, Floran B, Floran L, Aceves J, Young JM. Dopamine D(1) receptor facilitation of depolarization-induced release of gamma-amino-butyric acid in rat striatum is mediated by the cAMP/PKA pathway and involves P/Q-type calcium channels. Synapse. 2007;61:310–319. [Abstract] [Google Scholar]
- Arias-Montano J-A, Aceves J, Young JM. Carbachol-induced phosphoinositide metabolism in slices of rat substantia nigra pars reticulata. Mol Brain Res. 1993;19:233–236. [Abstract] [Google Scholar]
- Arnsten AF. Catecholamine regulation of the prefrontal cortex. J Psychopharmacol. 1997;11:151–162. [Abstract] [Google Scholar]
- Arnsten AF, Goldman-Rakic PS. Noise stress impairs prefrontal cortical cognitive function in monkeys: evidence for a hyperdopaminergic mechanism. Arch Gen Psychiatry. 1998;55:362–368. [Abstract] [Google Scholar]
- Arnt J, Hyttel J, Sanchez C. Partial and full dopamine D1 receptor agonists in mice and rats: relation between behavioural effects and stimulation of adenylate cyclase activity in vitro. Eur J Pharmacol. 1992;213:259–267. [Abstract] [Google Scholar]
- Bargas J, Howe A, Eberwine J, Cao Y, Surmeier DJ. Cellular and molecular characterization of Ca2+ currents in acutely isolated, adult rat neostriatal neurons. J Neurosci. 1994;14:6667–6686. [Europe PMC free article] [Abstract] [Google Scholar]
- Bathori G, Parolini I, Tombola F, Szabo I, Messina A, Oliva M, De P, V, Lisanti M, Sargiacomo M, Zoratti M. Porin is present in the plasma membrane where it is concentrated in caveolae and caveolae-related domains. J Biol Chem. 1999;274:29607–29612. [Abstract] [Google Scholar]
- Battaglia G, Norman AB, Hess EJ, Creese I. Functional recovery of D1 dopamine receptor-mediated stimulation of rat striatal adenylate cyclase activity following irreversible receptor modification by N-ethoxycarbonyl-2-ethoxy-1,2-dihydroquinoline (EEDQ): evidence for spare receptors. Neurosci Lett. 1986;69:290–295. [Abstract] [Google Scholar]
- Baufreton J, Garret M, Rivera A, de la CA, Gonon F, Dufy B, Bioulac B, Taupignon A. D5 (not D1) dopamine receptors potentiate burst-firing in neurons of the subthalamic nucleus by modulating an L-type calcium conductance. J Neurosci. 2003;23:816–825. [Europe PMC free article] [Abstract] [Google Scholar]
- Behrens UD, Wagner HJ. Localization of dopamine D1-receptors in vertebrate retinae. Neurochem Int. 1995;27:497–507. [Abstract] [Google Scholar]
- Beninger RJ, Nakonechny PL, Savina I. cAMP-dependent protein kinase and reward-related learning: intra-accumbens Rp-cAMPS blocks amphetamine-produced place conditioning in rats. Psychopharmacology (Berl) 2003;170:23–32. [Abstract] [Google Scholar]
- Bergson C, Levenson R, Goldman-Rakic PS, Lidow MS. Dopamine receptor-interacting proteins: the Ca(2+) connection in dopamine signaling. Trends Pharmacol Sci. 2003;24:486–492. [Abstract] [Google Scholar]
- Bergson C, Mrzljak L, Lidow MS, Goldman-Rakic PS, Levenson R. Characterization of subtype-specific antibodies to the human D5 dopamine receptor: Studies in primate brain and transfected mammalian cells. Proc Natl Acad Sci USA. 1995a;92:3468–3472. [Europe PMC free article] [Abstract] [Google Scholar]
- Bergson C, Mrzljak L, Smiley JF, Pappy M, Levenson R, Goldman-Rakic PS. Regional, cellular, and subcellular variations in the distribution of D1 and D5 dopamine receptors in primate brain. J Neurosci. 1995b;15:7821–7836. [Europe PMC free article] [Abstract] [Google Scholar]
- Bernard V, Laribi O, Levey AI, Bloch B. Subcellular redistribution of m2 muscarinic acetylcholine receptors in striatal interneurons in vivo after acute cholinergic stimulation. J Neurosci. 1998;18:10207–10218. [Europe PMC free article] [Abstract] [Google Scholar]
- Berridge MJ. Inositol trisphosphate and diacylglycerol as second messengers. Biochem J. 1984;220:345–360. [Europe PMC free article] [Abstract] [Google Scholar]
- Berridge MJ, Irvine RF. Inositol trisphosphate, a novel second messenger in cellular signal transduction. Nature. 1984;312:315–321. [Abstract] [Google Scholar]
- Besson MJ, Graybiel AM, Nastuk MA. [3H]SCH 23390 binding to D1 dopamine receptors in the basal ganglia of the cat and primate: delineation of striosomal compartments and pallidal and nigral subdivisions. Neuroscience. 1988;26:101–119. [Abstract] [Google Scholar]
- Beurel E, Jope RS. The paradoxical pro- and anti-apoptotic actions of GSK3 in the intrinsic and extrinsic apoptosis signaling pathways. Prog Neurobiol. 2006;79:173–189. [Europe PMC free article] [Abstract] [Google Scholar]
- Bhat RV, Haeberlein SL Budd, Avila J. Glycogen synthase kinase 3: a drug target for CNS therapies. J Neurochem. 2004;89:1313–1317. [Abstract] [Google Scholar]
- Bloch B, Dumartin B, Bernard V. In vivo regulation of intraneuronal trafficking of G protein-coupled receptors for neurotransmitters. Trends Pharmacol Sci. 1999;20:315–319. [Abstract] [Google Scholar]
- Blumberg AL, Wilson JW, Hieble JP. Neuroinhibitory effects of SKF85174, a novel dopamine receptor agonist. J Cardiovasc Pharmacol. 1985;7:723–732. [Abstract] [Google Scholar]
- Bouvier M. Oligomerization of G-protein-coupled transmitter receptors. Nat Rev Neurosci. 2001;2:274–286. [Abstract] [Google Scholar]
- Brami-Cherrier K, Valjent E, Garcia M, Pages C, Hipskind RA, Caboche J. Dopamine induces a PI3-kinase-independent activation of Akt in striatal neurons: a new route to cAMP response element-binding protein phosphorylation. J Neurosci. 2002;22:8911–8921. [Europe PMC free article] [Abstract] [Google Scholar]
- Brismar H, Agren M, Holtback U. beta-Adrenoceptor agonist sensitizes the dopamine-1 receptor in renal tubular cells. Acta Physiol Scand. 2002;175:333–340. [Abstract] [Google Scholar]
- Brunet A, Park J, Tran H, Hu LS, Hemmings BA, Greenberg ME. Protein kinase SGK mediates survival signals by phosphorylating the forkhead transcription factor FKHRL1 (FOXO3a) Mol Cell Biol. 2001;21:952–965. [Europe PMC free article] [Abstract] [Google Scholar]
- Bryson SE, Drew GM, Hall AS, Ball SG, Balmforth AJ. Characterization of the dopamine receptor expressed by rat glomerular mesangial cells in culture. Eur J Pharmacol. 1992;225:1–5. [Abstract] [Google Scholar]
- Cai JX, Arnsten AF. Dose-dependent effects of the dopamine D1 receptor agonists A77636 or SKF81297 on spatial working memory in aged monkeys. J Pharmacol Exp Ther. 1997;283:183–189. [Abstract] [Google Scholar]
- Caille I, Dumartin B, Bloch B. Ultrastructural localization of D1 dopamine receptor immunoreactivity in rat striatonigral neurons and its relation with dopaminergic innervation. Brain Res. 1996;730:17–31. [Abstract] [Google Scholar]
- Calabrese EJ. Hormesis: changing view of the dose-response, a personal account of the history and current status. Mutat Res. 2002;511:181–189. [Abstract] [Google Scholar]
- Calabrese EJ, Baldwin LA. Applications of hormesis in toxicology, risk assessment and chemotherapeutics. Trends Pharmacol Sci. 2002;23:331–337. [Abstract] [Google Scholar]
- Cantrell AR, Scheuer T, Catterall WA. Voltage-dependent neuromodulation of Na+ channels by D1-like dopamine receptors in rat hippocampal neurons. J Neurosci. 1999;19:5301–5310. [Europe PMC free article] [Abstract] [Google Scholar]
- Cardinaud B, Sugamori KS, Coudouel S, Vincent JD, Niznik HB, Vernier P. Early emergence of three dopamine D1 receptor subtypes in vertebrates. Molecular phylogenetic, pharmacological, and functional criteria defining D1A, D1B, and D1C receptors in European eel Anguilla anguilla. J Biol Chem. 1997;272:2778–2787. [Abstract] [Google Scholar]
- Carenzi A, Gillin JC, Guidotti A, Schwartz MA, Trabucchi M, Wyatt RJ. Dopamine-sensitive adenylyl cyclase in human caudate nucleus. A study in control subjects and schizophrenic patients. Arch Gen Psychiatry. 1975;32:1056–1059. [Abstract] [Google Scholar]
- Carman CV, Benovic JL. G-protein-coupled receptors: turn-ons and turn-offs. Curr Opin Neurobiol. 1998;8:335–344. [Abstract] [Google Scholar]
- Carman CV, Lisanti MP, Benovic JL. Regulation of G protein-coupled receptor kinases by caveolin. J Biol Chem. 1999;274:8858–8864. [Abstract] [Google Scholar]
- Centonze D, Grande C, Saulle E, Martin AB, Gubellini P, Pavon N, Pisani A, Bernardi G, Moratalla R, Calabresi P. Distinct roles of D1 and D5 dopamine receptors in motor activity and striatal synaptic plasticity. J Neurosci. 2003a;23:8506–8512. [Europe PMC free article] [Abstract] [Google Scholar]
- Centonze D, Grande C, Usiello A, Gubellini P, Erbs E, Martin AB, Pisani A, Tognazzi N, Bernardi G, Moratalla R, Borrelli E, Calabresi P. Receptor subtypes involved in the presynaptic and postsynaptic actions of dopamine on striatal interneurons. J Neurosci. 2003b;23:6245–6254. [Europe PMC free article] [Abstract] [Google Scholar]
- Charpentier S, Jarvie KR, Severynse DM, Caron MG, Tiberi M. Silencing of the constitutive activity of the dopamine D1B receptor. Reciprocal mutations between D1 receptor subtypes delineate residues underlying activation properties. J Biol Chem. 1996;271:28071–28076. [Abstract] [Google Scholar]
- Chen CJ, Vyas SJ, Eichberg J, Lokhandwala MF. Diminished phospholipase C activation by dopamine in spontaneously hypertensive rats. Hypertension. 1992;19:102–108. [Abstract] [Google Scholar]
- Choi WS, Machida CA, Ronnekleiv OK. Distribution of dopamine D1, D2, and D5 receptor mRNAs in the monkey brain: ribonuclease protection assay analysis. Brain Res Mol Brain Res. 1995;31:86–94. [Abstract] [Google Scholar]
- Ciliax BJ, Nash N, Heilman C, Sunahara R, Hartney A, Tiberi M, Rye DB, Caron MG, Niznik HB, Levey AI. Dopamine D(5) receptor immunolocalization in rat and monkey brain. Synapse. 2000;37:125–145. [Abstract] [Google Scholar]
- Civelli O, Bunzow JR, Grandy DK. Molecular diversity of the dopamine receptors. Annu Rev Pharmacol Toxicol. 1993;33:281–307. [Abstract] [Google Scholar]
- Clark EC, Baxter LR, Jr., Dure LS, Ackermann RF, Kemp GF, Bachus SE. Mammal-like striatal functions in Anolis. II. Distribution of dopamine D(1) and D(2) receptors, and a laminar pattern of basal ganglia sub-systems. Brain Behav Evol. 2000;56:249–258. [Abstract] [Google Scholar]
- Clifford JJ, Tighe O, Croke DT, Kinsella A, Sibley DR, Drago J, Waddington JL. Conservation of behavioural topography to dopamine D1-like receptor agonists in mutant mice lacking the D1A receptor implicates a D1-like receptor not coupled to adenylyl cyclase. Neuroscience. 1999;93:1483–1489. [Abstract] [Google Scholar]
- Cross DA, Alessi DR, Cohen P, Andjelkovich M, Hemmings BA. Inhibition of glycogen synthase kinase-3 by insulin mediated by protein kinase B. Nature. 1995;378:785–789. [Abstract] [Google Scholar]
- Cubitt AB, Brown BL, Dobson PR. Activation of dopamine receptors does not affect phosphoinositide turnover in NCB-20 cells. J Neurochem. 1987;49:183–188. [Abstract] [Google Scholar]
- De Camilli P, Emr SD, McPherson PS, Novick P. Phosphoinositides as regulators in membrane traffic. Science. 1996;271:1533–1539. [Abstract] [Google Scholar]
- de Chaffoy dC, Roevens P, Van Belle H. 1-Oleoyl-2-acetyl-glycerol (OAG) stimulates the formation of phosphatidylinositol 4-phosphate in intact human platelets. Biochem Biophys Res Commun. 1984;123:589–595. [Abstract] [Google Scholar]
- De Keyser J, De Waele M, Convents A, Ebinger G, Vauquelin G. Identification of D1-like dopamine receptors on human blood platelets. Life Sci. 1988a;42:1797–1806. [Abstract] [Google Scholar]
- De Keyser J, Dierckx R, Vanderheyden P, Ebinger G, Vauquelin G. D1 dopamine receptors in human putamen, frontal cortex and calf retina: differences in guanine nucleotide regulation of agonist binding and adenylate cyclase stimulation. Brain Res. 1988b;443:77–84. [Abstract] [Google Scholar]
- Dearry A, Gingrich JA, Falardeau P, Fremeau RTJ, Bates MD, Caron MG. Molecular cloning and expression of the gene for a human D1 dopamine receptor. Nature. 1990;347:72–76. [Abstract] [Google Scholar]
- Dekker LV, Parker PJ. Protein kinase C--a question of specificity. Trends Biochem Sci. 1994;19:73–77. [Abstract] [Google Scholar]
- Demchyshyn LL, McConkey F, Niznik HB. Dopamine D5 receptor agonist high affinity and constitutive activity profile conferred by carboxyl-terminal tail sequence. J Biol Chem. 2000;275:23446–23455. [Abstract] [Google Scholar]
- Demchyshyn LL, Sugamori KS, Lee FJS, Hamadanizadeh SA, Niznik HB. The dopamine D1D receptor. Cloning and characterization of three pharmacologically distinct D1-like receptors from Gallus domesticus. J Biol Chem. 1995;270:4005–4012. [Abstract] [Google Scholar]
- Deveney AM, Waddington JL. Pharmacological characterization of behavioural responses to SK&F 83959 in relation to ‘D1-like’ dopamine receptors not linked to adenylyl cyclase. Br J Pharmacol. 1995;116:2120–2126. [Europe PMC free article] [Abstract] [Google Scholar]
- Di M, V, Hill MP, Bisogno T, Crossman AR, Brotchie JM. Enhanced levels of endogenous cannabinoids in the globus pallidus are associated with a reduction in movement in an animal model of Parkinson’s disease. FASEB J. 2000;14:1432–1438. [Abstract] [Google Scholar]
- Domin J, Waterfield MD. Using structure to define the function of phosphoinositide 3-kinase family members. FEBS Lett. 1997;410:91–95. [Abstract] [Google Scholar]
- Drolet P, Bilodeau L, Chorvatova A, Laflamme L, Gallo-Payet N, Payet MD. Inhibition of the T-type Ca2+ current by the dopamine D1 receptor in rat adrenal glomerulosa cells: requirement of the combined action of the G betagamma protein subunit and cyclic adenosine 3′,5′-monophosphate. Mol Endocrinol. 1997;11:503–514. [Abstract] [Google Scholar]
- Dumartin B, Caille I, Gonon F, Bloch B. Internalization of D1 dopamine receptor in striatal neurons in vivo as evidence of activation by dopamine agonists. J Neurosci. 1998;18:1650–1661. [Europe PMC free article] [Abstract] [Google Scholar]
- Dyck LE. Effects of dopamine on phosphoinositide hydrolsis in slices of rat striatum and cortex. Neurochem Int. 1990;17:77–82. [Abstract] [Google Scholar]
- Eaton ME, Macias W, Youngs RM, Rajadhyaksha A, Dudman JT, Konradi C. L-type Ca2+ channel blockers promote Ca2+ accumulation when dopamine receptors are activated in striatal neurons. Brain Res Mol Brain Res. 2004;131:65–72. [Europe PMC free article] [Abstract] [Google Scholar]
- Emilien G, Maloteaux JM, Geurts M, Hoogenberg K, Cragg S. Dopamine receptors--physiological understanding to therapeutic intervention potential. Pharmacol Ther. 1999;84:133–156. [Abstract] [Google Scholar]
- Evans AM, Green KL. Effects of selective D1 and D2 dopamine agonists on cockroach salivary gland acinar cells in vitro. Br J Pharmacol. 1991;104:787–792. [Europe PMC free article] [Abstract] [Google Scholar]
- Evans AM, MacEwan DJ, Palmer S. The D1-like dopamine receptor of the cockroach salivary gland is coupled to phospholipase C in addition to adenylyl cyclase. Br J Pharmacol. 1991;102:212. [Google Scholar]
- Fabbrini G, Braun A, Mouradian MM, Tamminga CA, Chase TN. Dopamine D-1 receptor agonist stimulation of prolactin secretion in man. J Neural Transm. 1988;71:159–163. [Abstract] [Google Scholar]
- Felder CC, Albrecht F, Eisner GM, Jose PA. The signal transducer for the dopamine-1 regulated sodium transport in renal cortical brush border membrane vesicles. Am J Hypertens. 1990;3:47S–50S. [Abstract] [Google Scholar]
- Felder CC, Blecher M, Jose PA. Dopamine-1-mediated stimulation of phospholipase C activity in rat renal cortical membranes. J Biol Chem. 1989a;264:8739–8745. [Abstract] [Google Scholar]
- Felder CC, Pedro AJ, Axelrod J. The dopamine-1 agonist SKF82526 stimulates phospholipase-C activity independent of adenylate cyclase. J Pharmacol Exp Ther. 1989b;248:171–175. [Abstract] [Google Scholar]
- Ferreira-de-Almeida JA, Pereira-Leite L, Cavallotti C, Ricci A, Amenta F. Pharmacological characterization and autoradiographic localization of dopamine D1 receptors in the human umbilical artery. Eur J Pharmacol. 1993;234:209–214. [Abstract] [Google Scholar]
- Fienberg AA, Hiroi N, Mermelstein PG, Song W, Snyder GL, Nishi A, Cheramy A, O’Callaghan JP, Miller DB, Cole DG, Corbett R, Haile CN, Cooper DC, Onn SP, Grace AA, Ouimet CC, White FJ, Hyman SE, Surmeier DJ, Girault J, Nestler EJ, Greengard P. DARPP-32: regulator of the efficacy of dopaminergic neurotransmission. Science. 1998;281:838–842. [Abstract] [Google Scholar]
- Fiorentini C, Gardoni F, Spano P, Di Luca M, Missale C. Regulation of dopamine D1 receptor trafficking and desensitization by oligomerization with glutamate N-methyl-D-aspartate receptors. J Biol Chem. 2003;278:20196–20202. [Abstract] [Google Scholar]
- Fluckiger E, Muller EE, Thorner MO. Basic and Clinical Aspects of Neuroscience. Vol.2. Springer-Verlag; New York: 1987. Transmitter Molecules in the Brain; pp. 7–78. [Google Scholar]
- Frame S, Cohen P. GSK3 takes centre stage more than 20 years after its discovery. Biochem J. 2001;359:1–16. [Europe PMC free article] [Abstract] [Google Scholar]
- Franco R, Ferre S, Agnati L, Torvinen M, Gines S, Hillion J, Casado V, Lledo P, Zoli M, Lluis C, Fuxe K. Evidence for adenosine/dopamine receptor interactions: indications for heteromerization. Neuropsychopharmacology. 2000;23:S50–S59. [Abstract] [Google Scholar]
- Fride E. Endocannabinoids in the central nervous system--an overview. Prostaglandins Leukot Essent Fatty Acids. 2002;66:221–233. [Abstract] [Google Scholar]
- Friedman E, Jin LQ, Cai GP, Hollon TR, Drago J, Sibley DR, Wang HY. D1-like dopaminergic activation of phosphoinositide hydrolysis is independent of D1A dopamine receptors: evidence from D1A knockout mice. Mol Pharmacol. 1997;51:6–11. [Abstract] [Google Scholar]
- Friedman E, Wang HY. Receptor-mediated activation of G proteins is increased in postmortem brains of bipolar affective disorder subjects. J Neurochem. 1996;67:1145–1152. [Abstract] [Google Scholar]
- Galbiati F, Razani B, Lisanti MP. Emerging themes in lipid rafts and caveolae. Cell. 2001;106:403–411. [Abstract] [Google Scholar]
- Garau L, Govoni S, Stefanini E, Trabucchi M, Spano PF. Dopamine receptors: pharmacological and anatomical evidences indicate that two distinct dopamine receptor populations are present in rat striatum. Life Sci. 1978;23:1745–1750. [Abstract] [Google Scholar]
- Gardner CR, Cashin CH. Some aspects of monoamine function in the earthworm (Lumbricus terrestris) Neuropharmacology. 1975;14:483–500. [Abstract] [Google Scholar]
- George SR, Lee SP, Varghese G, Zeman PR, Seeman P, Ng GY, O’Dowd BF. A transmembrane domain-derived peptide inhibits D1 dopamine receptor function without affecting receptor oligomerization. J Biol Chem. 1998;273:30244–30248. [Abstract] [Google Scholar]
- George SR, O’Dowd BF, Lee SP. G-protein-coupled receptor oligomerization and its potential for drug discovery. Nat Rev Drug Discov. 2002;1:808–820. [Abstract] [Google Scholar]
- Ghosh D, Snyder SE, Watts VJ, Mailman RB, Nichols DE. 9-Dihydroxy-2,3,7,11b-tetrahydro-1H-naph[1,2,3-de]isoquinoline: a potent full dopamine D1 agonist containing a rigid-beta-phenyldopamine pharmacophore. J Med Chem. 1996;%19;39:549–555. [Abstract] [Google Scholar]
- Giambalvo CT, Wagner RL. Activation of D1 and D2 dopamine receptors inhibits protein kinase C activity in striatal synaptoneurosomes. J Neurochem. 1994;63:169–176. [Abstract] [Google Scholar]
- Gil M, Zhen X, Friedman E. Prenatal cocaine exposure alters glycogen synthase kinase-3beta (GSK3beta) pathway in select rabbit brain areas. Neurosci Lett. 2003;349:143–146. [Abstract] [Google Scholar]
- Gilmore JH, Watts VJ, Lawler CP, Noll EP, Nichols DE, Mailman RB. “Full” dopamine D1 agonists in human caudate: biochemical properties and therapeutic implications. Neuropharmacology. 1995;34:481–488. [Abstract] [Google Scholar]
- Gingrich JA, Caron MG. Recent advances in the molecular biology of dopamine receptors. Annu Rev Neurosci. 1993;16:299–321. [Abstract] [Google Scholar]
- Girbes AR, Van Veldhuisen DJ, Smit AJ. [New dopamine agonists in cardiovascular therapy] Presse Med. 1992;21:1287–1291. [Abstract] [Google Scholar]
- Giuffrida A, Parsons LH, Kerr TM, Rodriguez dF, Navarro M, Piomelli D. Dopamine activation of endogenous cannabinoid signaling in dorsal striatum. Nat Neurosci. 1999;2:358–363. [Abstract] [Google Scholar]
- Gnanalingham KK, Hunter AJ, Jenner P, Marsden CD. Stimulation of adenylate cyclase activity by benzazepine D-1 dopamine agonists with varying efficacies in the 6-hydroxydopamine lesioned rat--relationship to circling behaviour. Biochem Pharmacol. 1995;49:1185–1193. [Abstract] [Google Scholar]
- Gomes P, Soares-da-Silva P. Role of cAMP-PKA-PLC signaling cascade on dopamine-induced PKC-mediated inhibition of renal Na(+)-K(+)-ATPase activity. Am J Physiol Renal Physiol. 2002;282:F1084–F1096. [Abstract] [Google Scholar]
- Gomes P, Soares-da-Silva P. Dopamine acutely decreases type 3 Na(+)/H(+) exchanger activity in renal OK cells through the activation of protein kinases A and C signaling cascades. Eur J Pharmacol. 2004;488:51–59. [Abstract] [Google Scholar]
- Gotzes F, Balfanz S, Baumann A. Primary structure and functional characterization of a Drosophila dopamine receptor with high homology to human D1/5 receptors. Receptors Channels. 1994;2:131–141. [Abstract] [Google Scholar]
- Gouldson PR, Snell CR, Bywater RP, Higgs C, Reynolds CA. Domain swapping in G-protein coupled receptor dimers. Protein Eng. 1998;11:1181–1193. [Abstract] [Google Scholar]
- Grandy DK, Allen LJ, Zhang Y, Magenis RE, Civelli O. Chromosomal localization of three human D5 dopamine receptor genes. Genomics. 1992;13:968–973. [Abstract] [Google Scholar]
- Grandy DK, Zhang YA, Bouvier C, zhou qy, Johnson RA, Allen L, Buck K, Bunzow JR, Salon J, Civelli O. Multiple human D5 dopamine receptor genes: a functional receptor and two pseudogenes. Proc Natl Acad Sci U S A. 1991;88:9175–9179. [Europe PMC free article] [Abstract] [Google Scholar]
- Grandy DK, zhou qy, Allen L, Litt R, Magenis RE, Civelli O, Litt M. A human D1 dopamine receptor gene is located on chromosome 5 at q35.1 and identifies an EcoRI RFLP. Am J Hum Genet. 1990;47:828–834. [Europe PMC free article] [Abstract] [Google Scholar]
- Granon S, Passetti F, Thomas KL, Dalley JW, Everitt BJ, Robbins TW. Enhanced and impaired attentional performance after infusion of D1 dopaminergic receptor agents into rat prefrontal cortex. J Neurosci. 2000;20:1208–1215. [Europe PMC free article] [Abstract] [Google Scholar]
- Greengard P, Allen PB, Nairn AC. Beyond the dopamine receptor: the DARPP-32/protein phosphatase-1 cascade. Neuron. 1999;23:435–447. [Abstract] [Google Scholar]
- Grilli M, Nisoli E, Memo M, Missale C, Spano PF. Pharmacological characterization of D1 and D2 dopamine receptors in rat limbocortical areas. II. Dorsal hippocampus. Neurosci Lett. 1988;87:253–258. [Abstract] [Google Scholar]
- Gross RA, Uhler MD, Macdonald RL. The cyclic AMP-dependent protein kinase catalytic subunit selectively enhances calcium currents in rat nodose neurones. J Physiol. 1990;429:483–496. [Abstract] [Google Scholar]
- Gu WH, Yang S, Shi WX, Jin GZ, Zhen XC. Requirement of PSD-95 for dopamine D1 receptor modulating glutamate NR1a/NR2B receptor function. Acta Pharmacol Sin. 2007;28:756–762. [Abstract] [Google Scholar]
- Hague C, Uberti MA, Chen Z, Bush CF, Jones SV, Ressler KJ, Hall RA, Minneman KP. Olfactory receptor surface expression is driven by association with the beta2-adrenergic receptor. Proc Natl Acad Sci U S A. 2004;101:13672–13676. [Europe PMC free article] [Abstract] [Google Scholar]
- Han JY, Heo JS, Lee YJ, Lee JH, Taub M, Han HJ. Dopamine stimulates 45Ca2+ uptake through cAMP, PLC/PKC, and MAPKs in renal proximal tubule cells. J Cell Physiol. 2007;211:486–494. [Abstract] [Google Scholar]
- Han KA, Millar NS, Grotewiel MS, Davis RL. DAMB, a novel dopamine receptor expressed specifically in Drosophila mushroom bodies. Neuron. 1996;16:1127–1135. [Abstract] [Google Scholar]
- Hartman DS, Civelli O. Dopamine receptor diversity: molecular and pharmacological perspectives. Prog Drug Res. 1997;48:173–194. [Abstract] [Google Scholar]
- Hausdorff WP, Bouvier M, O’Dowd BF, Irons GP, Caron MG, Lefkowitz RJ. Phosphorylation sites on two domains of the beta 2-adrenergic receptor are involved in distinct pathways of receptor desensitization. J Biol Chem. 1989;264:12657–12665. [Abstract] [Google Scholar]
- Hebert TE, Loisel TP, Adam L, Ethier N, Onge SS, Bouvier M. Functional rescue of a constitutively desensitized beta2AR through receptor dimerization. Biochem J. 1998;330(Pt 1):287–293. [Europe PMC free article] [Abstract] [Google Scholar]
- Helms MN, Chen XJ, Ramosevac S, Eaton DC, Jain L. Dopamine regulation of amiloride-sensitive sodium channels in lung cells. Am J Physiol Lung Cell Mol Physiol. 2006;290:L710–L722. [Abstract] [Google Scholar]
- Hemmings HC, Jr., Greengard P. DARPP-32, a dopamine- and adenosine 3′:5′-monophosphate- regulated phosphoprotein: regional, tissue, and phylogenetic distribution. J Neurosci. 1986;6:1469–1481. [Europe PMC free article] [Abstract] [Google Scholar]
- Hernandez-Lopez S, Bargas J, Surmeier DJ, Reyes A, Galarraga E. D1 receptor activation enhances evoked discharge in neostriatal medium spiny neurons by modulating an L-type Ca2+ conductance. J Neurosci. 1997;17:3334–3342. [Europe PMC free article] [Abstract] [Google Scholar]
- Hersch SM, Ciliax BJ, Gutekunst CA, Rees HD, Heilman CJ, Yung KK, Bolam JP, Ince E, Yi H, Levey AI. Electron microscopic analysis of D1 and D2 dopamine receptor proteins in the dorsal striatum and their synaptic relationships with motor corticostriatal afferents. J Neurosci. 1995;15:5222–5237. [Europe PMC free article] [Abstract] [Google Scholar]
- Hersch SM, Gutekunst CA, Rees HD, Heilman CJ, Levey AI. Distribution of m1-m4 muscarinic receptor proteins in the rat striatum: light and electron microscopic immunocytochemistry using subtype-specific antibodies. J Neurosci. 1994;14:3351–3363. [Europe PMC free article] [Abstract] [Google Scholar]
- Hersi AI, Kitaichi K, Srivastava LK, Gaudreau P, Quirion R. Dopamine D-5 receptor modulates hippocampal acetylcholine release. Brain Res Mol Brain Res. 2000;76:336–340. [Abstract] [Google Scholar]
- Hill TD, Dean NM, Boynton AL. Inositol 1,3,4,5-tetrakisphosphate induces Ca2+ sequestration in rat liver cells. Science. 1988;242:1176–1178. [Abstract] [Google Scholar]
- Hollon TR, Bek MJ, Lachowicz JE, Ariano MA, Mezey E, Ramachandran R, Wersinger SR, Soares-da-Silva P, Liu ZF, Grinberg A, Drago J, Young WS, III, Westphal H, Jose PA, Sibley DR. Mice lacking D5 dopamine receptors have increased sympathetic tone and are hypertensive. J Neurosci. 2002;22:10801–10810. [Europe PMC free article] [Abstract] [Google Scholar]
- Holmes A, Hollon TR, Gleason TC, Liu Z, Dreiling J, Sibley DR, Crawley JN. Behavioral characterization of dopamine D5 receptor null mutant mice. Behav Neurosci. 2001;115:1129–1144. [Abstract] [Google Scholar]
- Homberg U. Neurotransmitters and neuropeptides in the brain of the locust. Microsc Res Tech. 2002;56:189–209. [Abstract] [Google Scholar]
- Horn AS, Korf J, Westerink BHC. The Neurobiology of Dopamine. Academic Press; London: 1979. [Google Scholar]
- Hotte M, Thuault S, Lachaise F, Dineley KT, Hemmings HC, Nairn AC, Jay TM. D1 receptor modulation of memory retrieval performance is associated with changes in pCREB and pDARPP-32 in rat prefrontal cortex. Behav Brain Res. 2006;171:127–133. [Abstract] [Google Scholar]
- Hunyady B, Palkovits M, Mozsik G, Molnar J, Feher K, Toth Z, Zolyomi A, Szalayova I, Key S, Sibley DR, Mezey E. Susceptibility of dopamine D5 receptor targeted mice to cysteamine. J Physiol Paris. 2001;95:147–151. [Abstract] [Google Scholar]
- Hussain T, Lokhandwala MF. Renal dopamine DA1 receptor coupling with G(S) and G(q/11) proteins in spontaneously hypertensive rats. Am J Physiol. 1997;272(Pt 2):F339–46. [Abstract] [Google Scholar]
- Jaber M, Robinson SW, Missale C, Caron MG. Dopamine receptors and brain function. Neuropharmacology. 1996;35:1503–1519. [Abstract] [Google Scholar]
- Jarvie KR, Tiberi M, Silvia C, Gingrich JA, Caron MG. Molecular cloning, stable expression and desensitization of the human dopamine D1b/D5 receptor. J Recept Res. 1993;13:573–590. [Abstract] [Google Scholar]
- Jiang N, Ou-Yang KQ, Cai SX, Hu YH, Xu ZL. Identification of human dopamine D1-like receptor agonist using a cell-based functional assay. Acta Pharmacol Sin. 2005;26:1181–1186. [Abstract] [Google Scholar]
- Jimerson DC. Role of dopamine mechanisms in the affective disorders. In: Meltzer HY, editor. Psychopharmacology: The Third Generation of Progress. Raven Press; New York: 1987. pp. 505–511. [Google Scholar]
- Jin LQ, Cai G, Wang HY, Smith C, Friedman E. Characterization of the phosphoinositide-linked dopamine receptor in a mouse hippocampal-neuroblastoma hybrid cell line. J Neurochem. 1998;71:1935–1943. [Abstract] [Google Scholar]
- Jin LQ, Wang HY, Friedman E. Stimulated D(1) dopamine receptors couple to multiple Galpha proteins in different brain regions. J Neurochem. 2001;78:981–990. [Abstract] [Google Scholar]
- Johansen PA, Hu X-T, White FJ. Relationship between D 1 dopamine receptors, adenylate cyclase, and the electrophysiological responses of rat nucleus accumbens neurons. J Neural Transm. 1991;86:97–113. [Abstract] [Google Scholar]
- Jope RS, Song L, Grimes CA, Pacheco MA, Dilley GE, Li X, Meltzer HY, Overholser JC, Stockmeier CA. Selective increases in phosphoinositide signaling activity and G protein levels in postmortem brain from subjects with schizophrenia or alcohol dependence. J Neurochem. 1998;70:763–771. [Abstract] [Google Scholar]
- Jope RS, Yuskaitis CJ, Beurel E. Glycogen synthase kinase-3 (GSK3): inflammation, diseases, and therapeutics. Neurochem Res. 2007;32:577–595. [Europe PMC free article] [Abstract] [Google Scholar]
- Jose PA, Yu PY, Yamaguchi I, Eisner GM, Mouradian MM, Felder CC, Felder RA. Dopamine D1 receptor regulation of phospholipase C. Hypertens Res. 1995;18(Suppl 1):S39–S42. [Abstract] [Google Scholar]
- Joseph SK, Hansen CA, Williamson JR. Inositol 1,3,4,5-tetrakisphosphate increases the duration of the inositol 1,4,5-trisphosphate-mediated Ca2+ transient. FEBS Lett. 1987;219:125–129. [Abstract] [Google Scholar]
- Juhasz JR, Hasbi A, Rashid AJ, So CH, George SR, O’Dowd BF. Mu-opioid receptor heterooligomer formation with the dopamine D1 receptor as directly visualized in living cells. Eur J Pharmacol. 2008;581:235–243. [Abstract] [Google Scholar]
- Kansra V, Chen C, Lokhandwala MF. Dopamine causes stimulation of protein kinase C in rat renal proximal tubules by activating dopamine D1 receptors. Eur J Pharmacol Mol Pharmacol. 1995;289:391–394. [Abstract] [Google Scholar]
- Karpa KD, Lidow MS, Pickering MT, Levenson R, Bergson C. N-linked glycosylation is required for plasma membrane localization of D5, but not D1, dopamine receptors in transfected mammalian cells. Mol Pharmacol. 1999;56:1071–1078. [Abstract] [Google Scholar]
- Kawaguchi Y, Wilson CJ, Augood SJ, Emson PC. Striatal interneurones: chemical, physiological and morphological characterization. Trends Neurosci. 1995;18:527–535. [Abstract] [Google Scholar]
- Keating C, Orchard I. The effects of dopamine agonists and antagonists on the secretory responses in the salivary glands of the locust (Locusta migratoria) J Insect Physiol. 2004;50:17–23. [Abstract] [Google Scholar]
- Kebabian JW, Calne DB. Multiple receptors for dopamine. Nature. 1979;277:93–96. [Abstract] [Google Scholar]
- Kehren V, Baumann A. Characterization of the 5′ regulatory region of the Drosophila Dmdop1 dopamine receptor-gene. Arch Insect Biochem Physiol. 2005;59:118–131. [Abstract] [Google Scholar]
- Khan WA, Blobe GC, Hannun YA. Arachidonic acid and free fatty acids as second messengers and the role of protein kinase C. Cell Signal. 1995;7:171–184. [Abstract] [Google Scholar]
- Kilts CD, Anderson CM, Ely TD, Mailman RB. The biochemistry and pharmacology of mesoamygdaloid dopamine neurons. Ann N Y Acad Sci. 1988;537:173–187. [Abstract] [Google Scholar]
- Kim L, Kimmel AR. GSK3 at the edge: regulation of developmental specification and cell polarization. Curr Drug Targets. 2006;7:1411–1419. [Abstract] [Google Scholar]
- Kirchheimer C, Mendez CF, Acquier A, Nowicki S. Role of 20-HETE in D1/D2 dopamine receptor synergism resulting in the inhibition of Na+-K+-ATPase activity in the proximal tubule. Am J Physiol Renal Physiol. 2007;292:F1435–F1442. [Abstract] [Google Scholar]
- Kishimoto A, Takai Y, Mori T, Kikkawa U, Nishizuka Y. Activation of calcium and phospholipid-dependent protein kinase by diacylglycerol, its possible relation to phosphatidylinositol turnover. J Biol Chem. 1980;255:2273–2276. [Abstract] [Google Scholar]
- Kong MM, Fan T, Varghese G, O’Dowd BF, George SR. Agonist-induced cell surface trafficking of an intracellularly sequestered D1 dopamine receptor homo-oligomer. Mol Pharmacol. 2006;70:78–89. [Abstract] [Google Scholar]
- Kozlov AP, Druzin MY, Kurzina NP, Malinina EP. The role of D1-dependent dopaminergic mechanisms of the frontal cortex in delayed responding in rats. Neurosci Behav Physiol. 2001;31:405–411. [Abstract] [Google Scholar]
- Kruse MS, Adachi S, Scott L, Holtback U, Greengard P, Aperia A, Brismar H. Recruitment of renal dopamine 1 receptors requires an intact microtubulin network. Pflugers Arch. 2003;445:534–539. [Abstract] [Google Scholar]
- Kubrusly RC, Ventura AL, Melo Reis RA, Serra GC, Yamasaki EN, Gardino PF, de Mello MC, de Mello FG. Norepinephrine acts as D1-dopaminergic agonist in the embryonic avian retina: late expression of beta1-adrenergic receptor shifts norepinephrine specificity in the adult tissue. Neurochem Int. 2007;50:211–218. [Abstract] [Google Scholar]
- Langley KC, Bergson C, Greengard P, Ouimet CC. Co-localization of the D1 dopamine receptor in a subset of DARPP-32-containing neurons in rat caudate-putamen. Neuroscience. 1997;78:977–983. [Abstract] [Google Scholar]
- Le Moine C, Normand E, Bloch B. Phenotypical characterization of the rat striatal neurons expressing the D1 dopamine receptor gene. Proc Natl Acad Sci U S A. 1991;88:4205–4209. [Europe PMC free article] [Abstract] [Google Scholar]
- Lee D, Huang W, Lim AT. Dopamine induces a biphasic modulation of hypothalamic ANF neurons: a ligand concentration-dependent effect involving D5 and D2 receptor interaction. Mol Psychiatry. 2000;5:39–48. [Abstract] [Google Scholar]
- Lee SP, So CH, Rashid AJ, Varghese G, Cheng R, Lanca AJ, O’Dowd BF, George SR. Dopamine D1 and D2 receptor Co-activation generates a novel phospholipase C-mediated calcium signal. J Biol Chem. 2004;279:35671–35678. [Abstract] [Google Scholar]
- Leonard SK, Anderson CM, Lachowicz JE, Schulz DW, Kilts CD, Mailman RB. Amygdaloid D1 receptors are not linked to stimulation of adenylate cyclase. Synapse. 2003;50:320–333. [Abstract] [Google Scholar]
- Levey AI, Hersch SM, Rye DB, Sunahara RK, Niznik HB, Kitt CA, Price DL, Maggio R, Brann MR, Ciliax BJ. Localization of D1 and D2 dopamine receptors in brain with subtype-specific antibodies. Proc Natl Acad Sci U S A. 1993;90:8861–8865. [Europe PMC free article] [Abstract] [Google Scholar]
- Li R, Chuang DM, Wyatt RJ, Kirch DG. Effect of chronic haloperidol treatment on dopamine-induced inositol phosphate formation in rat brain slices. Neurochem Res. 1994;19:673–678. [Abstract] [Google Scholar]
- Lidow MS, Koh PO, Arnsten AF. D1 dopamine receptors in the mouse prefrontal cortex: Immunocytochemical and cognitive neuropharmacological analyses. Synapse. 2003;47:101–108. [Abstract] [Google Scholar]
- Lindskog M, Svenningsson P, Fredholm BB, Greengard P, Fisone G. Activation of dopamine D2 receptors decreases DARPP-32 phosphorylation in striatonigral and striatopallidal projection neurons via different mechanisms. Neuroscience. 1999;88:1005–1008. [Abstract] [Google Scholar]
- Lisanti MP, Scherer PE, Tang Z, Sargiacomo M. Caveolae, caveolin and caveolin-rich membrane domains: a signaling hypothesis. Trends Cell Biol. 1994;4:231–235. [Abstract] [Google Scholar]
- Little JZ, Teyler TJ. GABAa receptor-mediated field potentials are enhanced in area CA1 following prenatal cocaine exposure. Brain Res Dev Brain Res. 1998;110:115–119. [Abstract] [Google Scholar]
- Liu F, Wan Q, Pristupa ZB, Yu XM, Wang YT, Niznik HB. Direct protein-protein coupling enables cross-talk between dopamine D5 and gamma-aminobutyric acid A receptors. Nature. 2000;403:274–280. [Abstract] [Google Scholar]
- Liu Y, Lasater EM. Calcium currents in turtle retinal ganglion cells. II. Dopamine modulation via a cyclic AMP-dependent mechanism. J Neurophysiol. 1994;71:743–752. [Abstract] [Google Scholar]
- Liu YF, Civelli O, zhou qy, Albert PR. Cholera toxin-sensitive 3′,5′-cyclic adenosine monophosphate and calcium signals of the human dopamine-D1 receptor: selective potentiation by protein kinase A. Mol Endocrinol. 1992;6:1815–1824. [Abstract] [Google Scholar]
- Lo CJ, Cryer HG, Maier RV. Prostaglandin E2 production by endotoxin-stimulated alveolar macrophages is regulated by phospholipase C pathways. J Trauma. 1996;40:557–562. [Abstract] [Google Scholar]
- Loomis-Husselbee JW, Cullen PJ, Dreikausen UE, Irvine RF, Dawson AP. Synergistic effects of inositol 1,3,4,5-tetrakisphosphate on inositol 2,4,5-triphosphate-stimulated Ca2+ release do not involve direct interaction of inositol 1,3,4,5-tetrakisphosphate with inositol triphosphate-binding sites. Biochem J. 1996;314(Pt 3):811–816. [Europe PMC free article] [Abstract] [Google Scholar]
- Losonczy MF, Davidson M, Davis KL. The dopamine hypothesis of schizophrenia. In: Meltzer HY, editor. Psychopharmacology: The Third Generation of Progress. Raven Press; New York: 1987. pp. 715–726. [Google Scholar]
- Mahan LC, Burch RM, Monsma FJ, Jr., Sibley DR. Expression of striatal D1 dopamine receptors coupled to inositol phosphate production and Ca2+ mobilization in Xenopus oocytes. Proc Natl Acad Sci U S A. 1990;87:2196–2200. [Europe PMC free article] [Abstract] [Google Scholar]
- Mailman RB, Schulz DW, Kilts CD, Lewis MH, Rollema H, Wyrick S. Multiple forms of the D1 dopamine receptor: its linkage to adenylate cyclase and psychopharmacological effects. Psychopharmacol Bull. 1986;22:593–598. [Abstract] [Google Scholar]
- Makihara Y, Okuda Y, Kawada C, Matsumoto M, Waddington JL, Koshikawa N, Tomiyama K. Differential involvement of cyclase- versus non-cyclase-coupled D1-like dopamine receptors in orofacial movement topography in mice: studies with SKF 83822. Neurosci Lett. 2007;415:6–10. [Abstract] [Google Scholar]
- Manning BD, Cantley LC. United at last: the tuberous sclerosis complex gene products connect the phosphoinositide 3-kinase/Akt pathway to mammalian target of rapamycin (mTOR) signaling. Biochem Soc Trans. 2003;31:573–578. [Abstract] [Google Scholar]
- Mannoury la Cour C, Vidal S, Pasteau V, Cussac D, Millan MJ. Dopamine D1 receptor coupling to Gs/olf and Gq in rat striatum and cortex: a scintillation proximity assay (SPA)/antibody-capture characterization of benzazepine agonists. Neuropharmacology. 2007;52:1003–1014. [Abstract] [Google Scholar]
- Mantyh CR, Vigna SR, Bollinger RR, Mantyh PW, Maggio JE, Pappas TN. Differential expression of substance P receptors in patients with Crohn’s disease and ulcerative colitis. Gastroenterology. 1995a;109:850–860. [Abstract] [Google Scholar]
- Mantyh PW, DeMaster E, Malhotra A, Ghilardi JR, Rogers SD, Mantyh CR, Liu H, Basbaum AI, Vigna SR, Maggio JE. Receptor endocytosis and dendrite reshaping in spinal neurons after somatosensory stimulation. Science. 1995b;268:1629–1632. [Abstract] [Google Scholar]
- Martin LP, Waszczak BL. D1 receptor stimulation enhances phosphoinositol (PI) hydrolysis in slices from rat substantia nigra (SN) Soc Neurosci Abstr. 1993;19:82. [Google Scholar]
- Matovcik LM, Hemmings HC, Jr., Kinder BK. DARPP-32 (dopamine and cAMP-regulated phosphoprotein, Mr 32, 000) is a membrane protein in the bovine parathyroid. FEBS Lett. 1995;364:67–74. [Abstract] [Google Scholar]
- Matsumoto K, Kohno S, Ojima K, Tezuka Y, Kadota S, Watanabe H. Effects of methylenechloride-soluble fraction of Japanese angelica root extract, ligustilide and butylidenephthalide, on pentobarbital sleep in group-housed and socially isolated mice. Life Sci. 1998;62:2073–2082. [Abstract] [Google Scholar]
- McHugh D, Sharp EM, Scheuer T, Catterall WA. Inhibition of cardiac L-type calcium channels by protein kinase C phosphorylation of two sites in the N-terminal domain. Proc Natl Acad Sci U S A. 2000;97:12334–12338. [Europe PMC free article] [Abstract] [Google Scholar]
- Meller E, Enz A, Goldstein M. Absence of receptor reserve at striatal dopamine receptors regulating cholinergic neuronal activity. Eur J Pharmacol. 1988;155:151–154. [Abstract] [Google Scholar]
- Memo M, Kleinman JE, Hanbauer I. Coupling of dopamine D1 recognition sites with adenylate cyclase in nuclei accumbens and caudatus of schizophrenics. Science. 1983;221:1304–1307. [Abstract] [Google Scholar]
- Mengod G, Niznik HB, Sunahara RK, Seeman P, O’Dowd BF, Palacios JM. Visualization of a dopamine D1 receptor mRNA in human and rat brain. BRESM. 1991;10:185–191. [Abstract] [Google Scholar]
- Ming Y, Zhang H, Long L, Wang F, Chen J, Zhen X. Modulation of Ca2+ signals by phosphatidylinositol-linked novel D1 dopamine receptor in hippocampal neurons. J Neurochem. 2006;98:1316–1323. [Abstract] [Google Scholar]
- Mogul DJ, Adams ME, Fox AP. Differential activation of adenosine receptors decreases N-type but potentiates P-type Ca2+ current in hippocampal CA3 neurons. Neuron. 1993;10:327–334. [Abstract] [Google Scholar]
- Momiyama T, Fukazawa Y. D1-like dopamine receptors selectively block P/Q-type calcium channels to reduce glutamate release onto cholinergic basal forebrain neurones of immature rats. J Physiol. 2007;580:103–117. [Abstract] [Google Scholar]
- Monsma FJ, Jr., Mahan LC, McVittie LD, Gerfen CR, Sibley DR. Molecular cloning and expression of a D1 dopamine receptor linked to adenylyl cyclase activation. Proc Natl Acad Sci U S A. 1990;87:6723–6727. [Europe PMC free article] [Abstract] [Google Scholar]
- Montague DM, Striplin CD, Overcash JS, Drago J, Lawler CP, Mailman RB. Quantification of D1B(D5) receptors in dopamine D1A receptor-deficient mice. Synapse. 2001;39:319–322. [Abstract] [Google Scholar]
- Mora-Ferrer C, Yazulla S, Studholme KM, Haak-Frendscho M. Dopamine D1-receptor immunolocalization in goldfish retina. J Comp Neurol. 1999;411:705–714. [Abstract] [Google Scholar]
- Muly EC, III, Szigeti K, Goldman-Rakic PS. D1 receptor in interneurons of macaque prefrontal cortex: distribution and subcellular localization. J Neurosci. 1998;18:10553–10565. [Europe PMC free article] [Abstract] [Google Scholar]
- Nakade S, Rhee SK, Hamanaka H, Mikoshiba K. Cyclic AMP-dependent phosphorylation of an immunoaffinity-purified homotetrameric inositol 1,4,5-trisphosphate receptor (type I) increases Ca2+ flux in reconstituted lipid vesicles. J Biol Chem. 1994;269:6735–6742. [Abstract] [Google Scholar]
- Nash SR, Godinot N, Caron MG. Cloning and characterization of the opossum kidney cell D1 dopamine receptor: expression of identical D1A and D1B dopamine receptor mRNAs in opossum kidney and brain. Mol Pharmacol. 1993;44:918–925. [Abstract] [Google Scholar]
- Nelson CS, Ikeda M, Gompf HS, Robinson ML, Fuchs NK, Yoshioka T, Neve KA, Allen CN. Regulation of melatonin 1a receptor signaling and trafficking by asparagine-124. Mol Endocrinol. 2001;15:1306–1317. [Abstract] [Google Scholar]
- Nguyen T, Bard J, Jin H, Taruscio D, Ward DC, Kennedy JL, Weinshank R, Seeman P, O’Dowd BF. Human dopamine D5 receptor pseudogenes. Gene. 1991a;109:211–218. [Abstract] [Google Scholar]
- Nguyen T, Sunahara R, Marchese A, Van Tol HH, Seeman P, O’Dowd BF. Transcription of a human dopamine D5 pseudogene. Biochem Biophys Res Commun. 1991b;181:16–21. [Abstract] [Google Scholar]
- Nicola SM, Kombian SB, Malenka RC. Psychostimulants depress excitatory synaptic transmission in the nucleus accumbens via presynaptic D1-like dopamine receptors. J Neurosci. 1996;16:1591–1604. [Europe PMC free article] [Abstract] [Google Scholar]
- Nieuwenhuys R. Chemoarchitecture of the Brain. Springer-Verlag; New York: 1985. [Google Scholar]
- Nishi A, Snyder GL, Greengard P. Bidirectional regulation of DARPP-32 phosphorylation by dopamine. J Neurosci. 1997;17:8147–8155. [Europe PMC free article] [Abstract] [Google Scholar]
- Nishizuka Y. Studies and prospectives of protein kinase C in signal transduction. Nippon Ketsueki Gakkai Zasshi. 1988;51:1321–1326. [Abstract] [Google Scholar]
- Nisoli E, Grilli M, Memo M, Missale C, Spano PF. Pharmacological characterization of D1 and D2 dopamine receptors in rat limbocortical areas. I. Frontal cortex. Neurosci Lett. 1988;87:247–252. [Abstract] [Google Scholar]
- Niznik HB, Jarvie KR, Bzowej NH, Seeman P, Garlick RK, Miller JJ, Jr., Baindur N, Neumeyer JL. Photoaffinity labeling of dopamine D1 receptors. Biochemistry. 1988;27:7594–7599. [Abstract] [Google Scholar]
- Nomura M, Kaji A, Ma WY, Zhong S, Liu G, Bowden GT, Miyamoto KI, Dong Z. Mitogen- and stress-activated protein kinase 1 mediates activation of Akt by ultraviolet B irradiation. J Biol Chem. 2001;276:25558–25567. [Abstract] [Google Scholar]
- Nowicki S, Kruse MS, Brismar H, Aperia A. Dopamine-induced translocation of protein kinase C isoforms visualized in renal epithelial cells. Am J Physiol Cell Physiol. 2000;279:C1812–C1818. [Abstract] [Google Scholar]
- O’Connell DP, Botkin SJ, Ramos SI, Sibley DR, Ariano MA, Felder RA, Carey RM. Localization of dopamine D1A receptor protein in rat kidneys. Am J Physiol. 1995;268:F1185–F1197. [Abstract] [Google Scholar]
- O’Dowd BF. Structures of dopamine receptors. J Neurochem. 1993;60:804–816. [Abstract] [Google Scholar]
- O’Dowd BF, Ji X, Alijaniaram M, Rajaram RD, Kong MM, Rashid A, Nguyen T, George SR. Dopamine receptor oligomerization visualized in living cells. J Biol Chem. 2005;280:37225–37235. [Abstract] [Google Scholar]
- O’Sullivan GJ, Clifford JJ, Tomiyama K, Koshikawa N, Drago J, Sibley DR, Croke DT, Waddington JL. D1-like dopamine receptor-mediated function in congenic mutants with D1 vs. D5 receptor “knockout” J Recept Signal Transduct Res. 2004;24:107–116. [Abstract] [Google Scholar]
- O’Sullivan GJ, Kinsella A, Sibley DR, Tighe O, Croke DT, Waddington JL. Ethological resolution of behavioural topography and D1-like versus D2-like agonist responses in congenic D5 dopamine receptor mutants: identification of D5:D2-like interactions. Synapse. 2005;55:201–211. [Abstract] [Google Scholar]
- Obejero-Paz CA, Auslender M, Scarpa A. PKC activity modulates availability and long openings of L-type Ca2+ channels in A7r5 cells. Am J Physiol. 1998;275:C535–C543. [Abstract] [Google Scholar]
- Onali P, Olianas MC, Gessa GL. Selective blockade of dopamine D-1 receptors by SCH23390 discloses striatal dopamine D-2 receptors mediating the inhibition of adenylate cyclase in rats. Eur J Pharmacol. 1984;99:127–128. [Abstract] [Google Scholar]
- Orr GL, Gole JW, Notman HJ, Downer RG. Pharmacological characterisation of the dopamine-sensitive adenylate cyclase in cockroach brain: evidence for a distinct dopamine receptor. Life Sci. 1987;41:2705–2715. [Abstract] [Google Scholar]
- Ostrom RS, Post SR, Insel PA. Stoichiometry and compartmentation in G protein-coupled receptor signaling: implications for therapeutic interventions involving G(s) J Pharmacol Exp Ther. 2000;294:407–412. [Abstract] [Google Scholar]
- Ouimet CC, Greengard P. Distribution of DARPP-32 in the basal ganglia: an electron microscopic study. J Neurocytol. 1990;19:39–52. [Abstract] [Google Scholar]
- Ouimet CC, Miller PE, Hemmings HC, Jr., Walaas SI, Greengard P. DARPP-32, a dopamine- and adenosine 3′:5′-monophosphate-regulated phosphoprotein enriched in dopamine-innervated brain regions. III. Immunocytochemical localization. J Neurosci. 1984;4:111–124. [Europe PMC free article] [Abstract] [Google Scholar]
- Ozono R, O’Connell DP, Wang ZQ, Moore AF, Sanada H, Felder RA, Carey RM. Localization of the dopamine D1 receptor protein in the human heart and kidney. Hypertension. 1997;30:725–729. [Abstract] [Google Scholar]
- Ozono R, Oconnell DP, Vaughan C, Botkin SJ, Walk SF, Felder RA, Carey RM. Expression of the subtype 1a dopamine receptor in the rat heart. Hypertension. 1996;27(Part 2):693–703. [Abstract] [Google Scholar]
- Pacheco MA, Jope RS. Comparison of [3H]phosphatidylinositol and [3H]phosphatidylinositol 4,5-bisphosphate hydrolysis in postmortem human brain membranes and characterization of stimulation by dopamine D1 receptors. J Neurochem. 1997;69:639–644. [Abstract] [Google Scholar]
- Panchalingam S, Undie AS. Optimized binding of [35S]GTPγS to Gq-like proteins stimulated with dopamine D1-like receptor agonists. Neurochem Res. 2000;25:759–767. [Abstract] [Google Scholar]
- Panchalingam S, Undie AS. SKF83959 exhibits biochemical agonism by stimulating phosphoinositide hydrolysis and [35S]GTPγS binding in rat and monkey brain. Neuropharmacology. 2001;40:826–837. [Abstract] [Google Scholar]
- Panchalingam S, Undie AS. Physicochemical modulation of agonist-induced [35s]GTPgammaS binding: implications for coexistence of multiple functional conformations of dopamine D1-like receptors. J Recept Signal Transduct Res. 2005;25:125–146. [Abstract] [Google Scholar]
- Pap M, Cooper GM. Role of glycogen synthase kinase-3 in the phosphatidylinositol 3-Kinase/Akt cell survival pathway. J Biol Chem. 1998;273:19929–19932. [Abstract] [Google Scholar]
- Paspalas CD, Goldman-Rakic PS. Microdomains for dopamine volume neurotransmission in primate prefrontal cortex. J Neurosci. 2004;24:5292–5300. [Europe PMC free article] [Abstract] [Google Scholar]
- Pedrosa R, Gomes P, Soares-da-Silva P. Distinct signaling cascades downstream to Gsalpha coupled dopamine D1-like NHE3 inhibition in rat and opossum renal epithelial cells. Cell Physiol Biochem. 2004;14:91–100. [Abstract] [Google Scholar]
- Piomelli D, Pilon C, Giros B, Sokoloff P, Martres M-P, Schwartz J-C. Dopamine activation of the arachidonic acid cascade as a basis for D1/D2 receptor synergism. Nature. 1991;353:164–167. [Abstract] [Google Scholar]
- Pizzi M, Da Prada M, Valerio A, Memo M, Spano PF, Haefely WE. Dopamine D2 receptor stimulation inhibits inositol phosphate generating system in rat striatal slices. Brain Res. 1988;456:235–240. [Abstract] [Google Scholar]
- Pizzi M, DAgostini F, Da Prada M, Spano PF, Haefely WE. Dopamine D2 receptor stimulation decreases the inositol trisphosphate level of rat striatal slices. Eur J Pharmacol. 1987;136:263–264. [Abstract] [Google Scholar]
- Probst WC, Snyder LA, Schuster DI, Brosius J, Sealfon SC. Sequence alignment of the G-protein coupled receptor superfamily. DNA Cell Biol. 1992;11:1–20. [Abstract] [Google Scholar]
- Rashid AJ, O’Dowd BF, Verma V, George SR. Neuronal Gq/11-coupled dopamine receptors: an uncharted role for dopamine. Trends Pharmacol Sci. 2007a;28:551–555. [Abstract] [Google Scholar]
- Rashid AJ, So CH, Kong MM, Furtak T, El-Ghundi M, Cheng R, O’Dowd BF, George SR. D1-D2 dopamine receptor heterooligomers with unique pharmacology are coupled to rapid activation of Gq/11 in the striatum. Proc Natl Acad Sci U S A. 2007b;104:654–659. [Europe PMC free article] [Abstract] [Google Scholar]
- Razani B, Rubin CS, Lisanti MP. Regulation of cAMP-mediated signal transduction via interaction of caveolins with the catalytic subunit of protein kinase A. J Biol Chem. 1999;274:26353–26360. [Abstract] [Google Scholar]
- Reale V, Hannan F, Hall LM, Evans PD. Agonist-specific coupling of a cloned Drosophila melanogaster D1-like dopamine receptor to multiple second messenger pathways by synthetic agonists. J Neurosci. 1997;17:6545–6553. [Europe PMC free article] [Abstract] [Google Scholar]
- Rivera A, Alberti I, Martin AB, Narvaez JA, de la CA, Moratalla R. Molecular phenotype of rat striatal neurons expressing the dopamine D5 receptor subtype. Eur J Neurosci. 2002;16:2049–2058. [Abstract] [Google Scholar]
- Rosengarten H, Friedhoff AJ. A phosphoinositide-linked dopamine D1 receptor mediates repetitive jaw movements in rats. Biol Psychiatry. 1998;44:1178–1184. [Abstract] [Google Scholar]
- Roth RH, Wolf ME, Deutch AY. Neurochemistry of midbrain dopamine systems. In: Meltzer HY, editor. Psychopharmacology: The Third Generation of Progress. Raven Press; New York: 1987. pp. 81–94. [Google Scholar]
- Rubinstein JE, Hitzemann RJ. Further evidence against the coupling of dopamine receptors to phosphoinositide hydrolysis in rat striatum. Biochem Pharmacol. 1990;39:1965–1970. [Abstract] [Google Scholar]
- Ruskin DN, Rawji SS, Walters JR. Effects of full D1 dopamine receptor agonists on firing rates in the globus pallidus and substantia nigra pars compacta in vivo: tests for D1 receptor selectivity and comparisons to the partial agonist SKF 38393. J Pharmacol Exp Ther. 1998;286:272–281. [Abstract] [Google Scholar]
- Ryman-Rasmussen JP, Griffith A, Oloff S, Vaidehi N, Brown JT, Goddard WA, III, Mailman RB. Functional selectivity of dopamine D1 receptor agonists in regulating the fate of internalized receptors. Neuropharmacology. 2007;52:562–575. [Europe PMC free article] [Abstract] [Google Scholar]
- Sadowski L, Pilecka I, Miaczynska M. Signaling from endosomes: Location makes a difference. Exp Cell Res. 2008 [Abstract] [Google Scholar]
- Sahu A, Tyeryar KR, Vongtau HO, Sibley DR, Undieh AS. D5 dopamine receptors are required for dopaminergic activation of phospholipase C. Mol Pharmacol. 2009;75:447–453. [Europe PMC free article] [Abstract] [Google Scholar]
- Saller CF, Salama AI. D-1 dopamine receptor stimulation elevates plasma prolactin levels. Eur J Pharmacol. 1986;122:139–142. [Abstract] [Google Scholar]
- Sargiacomo M, Sudol M, Tang Z, Lisanti MP. Signal transducing molecules and glycosyl-phosphatidylinositol-linked proteins form a caveolin-rich insoluble complex in MDCK cells. J Cell Biol. 1993;122:789–807. [Europe PMC free article] [Abstract] [Google Scholar]
- Sauer JR, McSwain JL, Essenberg RC. Cell membrane receptors and regulation of cell function in ticks and blood-sucking insects. Int J Parasitol. 1994;24:33–52. [Abstract] [Google Scholar]
- Sawaguchi T, Goldman-Rakic PS. D1 dopamine receptors in prefrontal cortex: involvement in working memory. Science. 1991;251:947–950. [Abstract] [Google Scholar]
- Sawaguchi T, Goldman-Rakic PS. The role of D1-dopamine receptor in working memory: local injections of dopamine antagonists into the prefrontal cortex of rhesus monkeys performing an oculomotor delayed-response task. J Neurophysiol. 1994;71:515–528. [Abstract] [Google Scholar]
- Scheid MP, Woodgett JR. PKB/AKT: functional insights from genetic models. Nat Rev Mol Cell Biol. 2001;2:760–768. [Abstract] [Google Scholar]
- Schetz JA, Kim OJ, Sibley DR. Pharmacological characterization of mammalian D1 and D2 dopamine receptors expressed in Drosophila Schneider-2 cells. J Recept Signal Transduct Res. 2003;23:99–109. [Europe PMC free article] [Abstract] [Google Scholar]
- Schmidt SP, Essenberg RC, Sauer JR. Evidence for a D1 dopamine receptor in the salivary glands of Amblyomma americanum (L.) J Cyclic Nucleotide Res. 1981;7:375–384. [Abstract] [Google Scholar]
- Schnabel R, Metzger M, Jiang S, Hemmings HC, Jr., Greengard P, Braun K. Localization of dopamine D1 receptors and dopaminoceptive neurons in the chick forebrain. J Comp Neurol. 1997;388:146–168. [Abstract] [Google Scholar]
- Schoors DF, Vauquelin GP, De Vos H, Smets G, Velkeniers B, Vanhaelst L, Dupont AG. Identification of a D1 dopamine receptor, not linked to adenylate cyclase, on lactotroph cells. Br J Pharmacol. 1991;103:1928–1934. [Europe PMC free article] [Abstract] [Google Scholar]
- Schutte M. [125I]SCH 23982, a new tool for rapid visualization of dopaminergic neurons in lower vertebrate retinas. Neurosci Lett. 1991;121:29–33. [Abstract] [Google Scholar]
- Seamans JK, Floresco SB, Phillips AG. D1 receptor modulation of hippocampal-prefrontal cortical circuits integrating spatial memory with executive functions in the rat. J Neurosci. 1998;18:1613–1621. [Europe PMC free article] [Abstract] [Google Scholar]
- Sedvall G, Farde L, Barnett A, Hall H, Halldin C. 11C-SCH 39166, a selective ligand for visualization of dopamine-D1 receptor binding in the monkey brain using PET. Psychopharmacology (Berl) 1991;103:150–153. [Abstract] [Google Scholar]
- Seeman P. Brain dopamine receptors. Pharmacol Rev. 1980;32:229–313. [Abstract] [Google Scholar]
- Seeman P, Guan HC, Civelli O, Van Tol HH, Sunahara RK, Niznik HB. The cloned dopamine D2 receptor reveals different densities for dopamine receptor antagonist ligands. Implications for human brain positron emission tomography. Eur J Pharmacol. 1992;227:139–146. [Abstract] [Google Scholar]
- Seeman P, Van Tol HHM. Dopamine receptor pharmacology. Trends Pharmacol Sci. 1994;15:264–270. [Abstract] [Google Scholar]
- Sesack SR, Carr DB, Omelchenko N, Pinto A. Anatomical substrates for glutamate-dopamine interactions: evidence for specificity of connections and extrasynaptic actions. Ann N Y Acad Sci. 2003;1003:36–52. [Abstract] [Google Scholar]
- Shahedi M, Laborde K, Bussieres L, Dechaux M, Sachs C. Protein kinase C activation causes inhibition of Na/K-ATPase activity in Madin-Darby canine kidney epithelial (MDCK) cells. Pflugers Arch. 1992;420:269–274. [Abstract] [Google Scholar]
- Shamsuddin AM. Metabolism and cellular functions of IP6: a review. Anticancer Res. 1999;19:3733–3736. [Abstract] [Google Scholar]
- Shaul PW, Anderson RG. Role of plasmalemmal caveolae in signal transduction. Am J Physiol. 1998;275:L843–L851. [Abstract] [Google Scholar]
- Shimizu T, Wolfe LS. Arachidonic acid cascade and signal transduction. J Neurochem. 1990;55:1–15. [Abstract] [Google Scholar]
- Shpakov AO, Shpakova EA, Kuznetsova LA, Plesneva SA, Pertseva MN. [Structural and functional characteristics of the adenylyl cyclase signaling system regulated by biogenic amines and peptide hormones in the muscle of a worm Lumbricus terrestris] Zh Evol Biokhim Fiziol. 2008;44:467–475. [Abstract] [Google Scholar]
- Sibley DR. New insights into dopaminergic receptor function using antisense and genetically altered animals. Annu Rev Pharmacol Toxicol. 1999;39:313–41. 313-341. [Abstract] [Google Scholar]
- Sibley DR, Monsma FJ, Jr., Shen Y. Molecular neurobiology of dopaminergic receptors. Int Rev Neurobiol. 1993;35:391–415. [Abstract] [Google Scholar]
- Sidhu A, Banta M, Uh M, Shin Y. Photoaffinity labeling of D1 and D5 dopamine receptors. Neuroscience. 1998a;82:1095–1101. [Abstract] [Google Scholar]
- Sidhu A, Fishman PH. Identification and characterization of functional D1 dopamine receptors in a human neuroblastoma cell line. Biochem Biophys Res Commun. 1990;166:574–579. [Abstract] [Google Scholar]
- Sidhu A, Kimura K, Uh M, White BH, Patel S. Multiple coupling of human D5 dopamine receptors to guanine nucleotide binding proteins Gs and Gz. J Neurochem. 1998b;70:2459–2467. [Abstract] [Google Scholar]
- Sidhu A, Niznik HB. Coupling of dopamine receptor subtypes to multiple and diverse G proteins. Int J Dev Neurosci. 2000;18:669–677. [Abstract] [Google Scholar]
- Simons K, Ikonen E. Functional rafts in cell membranes. Nature. 1997;387:569–572. [Abstract] [Google Scholar]
- Simpson CS, Morris BJ. Induction of c-fos and zif/268 gene expression in rat striatal neurons, following stimulation of D1-like dopamine receptors, involves protein kinase A and protein kinase C. Neuroscience. 1995;68:97–106. [Abstract] [Google Scholar]
- Smart EJ, Ying YS, Mineo C, Anderson RG. A detergent-free method for purifying caveolae membrane from tissue culture cells. Proc Natl Acad Sci U S A. 1995;92:10104–10108. [Europe PMC free article] [Abstract] [Google Scholar]
- Smiley JF, Levey AI, Ciliax BJ, Goldman-Rakic PS. D1 dopamine receptor immunoreactivity in human and monkey cerebral cortex: predominant and extrasynaptic localization in dendritic spines. Proc Natl Acad Sci U S A. 1994;91:5720–5724. [Europe PMC free article] [Abstract] [Google Scholar]
- So CH, Varghese G, Curley KJ, Kong MM, Alijaniaram M, Ji X, Nguyen T, O’Dowd BF, George SR. D1 and D2 dopamine receptors form heterooligomers and cointernalize after selective activation of either receptor. Mol Pharmacol. 2005;68:568–578. [Abstract] [Google Scholar]
- So CH, Verma V, Alijaniaram M, Cheng R, Rashid AJ, O’Dowd BF, George SR. Calcium Signaling by Dopamine D5-D2 Receptor Heterooligomers Occurs by a Mechanism Distinct From That For Dopamine D1-D2 Receptor Heterooligomers. Mol Pharmacol. 2009 [Europe PMC free article] [Abstract] [Google Scholar]
- Soares HC, Melo Reis RA, de Mello FG, Ventura AL, Kurtenbach E. Differential expression of D(1A) and D(1B) dopamine receptor mRNAs in the developing avian retina. J Neurochem. 2000;75:1071–1075. [Abstract] [Google Scholar]
- Solinas M, Goldberg SR, Piomelli D. The endocannabinoid system in brain reward processes. Br J Pharmacol. 2008;154:369–383. [Europe PMC free article] [Abstract] [Google Scholar]
- Song KS, Li S, Okamoto T, Quilliam LA, Sargiacomo M, Lisanti MP. Co-purification and direct interaction of Ras with caveolin, an integral membrane protein of caveolae microdomains. Detergent-free purification of caveolae microdomains. J Biol Chem. 1996;271:9690–9697. [Abstract] [Google Scholar]
- Spano PF, Govoni S, Trabucchi M. Studies on the pharmacological properties of dopamine receptors in various areas of the central nervous system. Adv Biochem Psychopharmacol. 1978;19:155–165. [Abstract] [Google Scholar]
- Stella N, Piomelli D. Receptor-dependent formation of endogenous cannabinoids in cortical neurons. Eur J Pharmacol. 2001;425:189–196. [Abstract] [Google Scholar]
- Sternini C, Spann M, Anton B, Keith DE, Jr., Bunnett NW, von Zastrow M, Evans C, Brecha NC. Agonist-selective endocytosis of mu opioid receptor by neurons in vivo. Proc Natl Acad Sci U S A. 1996;93:9241–9246. [Europe PMC free article] [Abstract] [Google Scholar]
- Stoof JC, Kebabian JW. Opposing roles for D-1 and D-2 dopamine receptors in efflux of cyclic AMP from rat neostriatum. Nature. 1981;294:366–368. [Abstract] [Google Scholar]
- Stoof JC, Kebabian JW. Independent in vitro regulation by the D-2 receptor of dopamine-stimulated efflux of cyclic AMP and K+-stimulated release of acetylcholine from rat neostriatum. Brain Res. 1982;250:263–270. [Abstract] [Google Scholar]
- Sugamori KS, Demchyshyn LL, McConkey F, Forte MA, Niznik HB. A primordial dopamine D1-like adenylyl cyclase-linked receptor from Drosophila melanogaster displaying poor affinity for benzazepines. FEBS Lett. 1995;362:131–138. [Abstract] [Google Scholar]
- Sugamori KS, Hamadanizadeh SA, Scheideler MA, Hohlweg R, Vernier P, Niznik HB. Functional differentiation of multiple dopamine D1-like receptors by NNC 01-0012. J Neurochem. 1998;71:1685–1693. [Abstract] [Google Scholar]
- Sugiura T, Kishimoto S, Oka S, Gokoh M. Biochemistry, pharmacology and physiology of 2-arachidonoylglycerol, an endogenous cannabinoid receptor ligand. Prog Lipid Res. 2006;45:405–446. [Abstract] [Google Scholar]
- Sugiura T, Kondo S, Sukagawa A, Nakane S, Shinoda A, Itoh K, Yamashita A, Waku K. 2-Arachidonoylglycerol: a possible endogenous cannabinoid receptor ligand in brain. Biochem Biophys Res Commun. 1995;215:89–97. [Abstract] [Google Scholar]
- Sui L, Wang J, Li BM. Role of the phosphoinositide 3-kinase-Akt-mammalian target of the rapamycin signaling pathway in long-term potentiation and trace fear conditioning memory in rat medial prefrontal cortex. Learn Mem. 2008;15:762–776. [Abstract] [Google Scholar]
- Sun Z, Reiner A. Localization of dopamine D1A and D1B receptor mRNAs in the forebrain and midbrain of the domestic chick. J Chem Neuroanat. 2000;19:211–224. [Abstract] [Google Scholar]
- Sunahara RK, Guan H-C, O’Dowd BF, Seeman P, Laurier LG, Ng G, Torchia J, Van Tol HHM, Niznik HB. Cloning of the gene for a human dopamine D5 receptor with higher affinity for dopamine than D1. Nature. 1991;350:614–619. [Abstract] [Google Scholar]
- Sunahara RK, Niznik HB, Weiner DM, Stormann TM, Brann MR, Kennedy JL, Gelernter JE, Rozmahel R, Yang Y, Isreal Y, Seeman P, O’Dowd BF. Human dopamine D1 receptor encoded by an intronless gene on chromosome 5. Nature. 1990;347:80–83. [Abstract] [Google Scholar]
- Surmeier DJ, Bargas J, Hemmings HC, Jr., Nairn AC, Greengard P. Modulation of calcium currents by a D1 dopaminergic protein kinase/phosphatase cascade in rat neostriatal neurons. Neuron. 1995;14:385–397. [Abstract] [Google Scholar]
- Takahashi N, Nagai Y, Ueno S, Saeki Y, Yanagihara T. Human peripheral blood lymphocytes express D5 dopamine receptor gene and transcribe the two pseudogenes. FEBS Lett. 1992;314:23–25. [Abstract] [Google Scholar]
- Takai Y, Kikkawa U, Kaibuchi K, Nishizuka Y. Membrane phospholipid metabolism and signal transduction for protein phosphorylation. Adv Cyclic Nucleotide Protein Phosphorylation Res. 1984;18:119–158. [Abstract] [Google Scholar]
- Tang TS, Tu H, Wang Z, Bezprozvanny I. Modulation of type 1 inositol (1,4,5)-trisphosphate receptor function by protein kinase a and protein phosphatase 1alpha. J Neurosci. 2003;23:403–415. [Europe PMC free article] [Abstract] [Google Scholar]
- Thorne RG, Lakkaraju A, Rodriguez-Boulan E, Nicholson C. In vivo diffusion of lactoferrin in brain extracellular space is regulated by interactions with heparan sulfate. Proc Natl Acad Sci U S A. 2008;105:8416–8421. [Europe PMC free article] [Abstract] [Google Scholar]
- Thorne RG, Nicholson C. In vivo diffusion analysis with quantum dots and dextrans predicts the width of brain extracellular space. Proc Natl Acad Sci U S A. 2006;103:5567–5572. [Europe PMC free article] [Abstract] [Google Scholar]
- Tiberi M, Caron MG. High agonist-independent activity is a distinguishing feature of the dopamine D1B receptor subtype. J Biol Chem. 1994;269:27925–27931. [Abstract] [Google Scholar]
- Tiberi M, Jarvie KR, Silvia C, Falardeau P, Gingrich JA, Godinot N, Bertrand L, Yang-Feng TL, Fremeau RTJ, Caron MG. Cloning, molecular characterization, and chromosonal assignment of a gene encoding a second D1 dopamine receptor subtype: Differential expression pattern in rat brain compared with the D1A receptor. Proc Natl Acad Sci U S A. 1991;88:7491–7495. [Europe PMC free article] [Abstract] [Google Scholar]
- Toker A, Cantley LC. Signaling through the lipid products of phosphoinositide-3-OH kinase. Nature. 1997;387:673–676. [Abstract] [Google Scholar]
- Toker A, Meyer M, Reddy KK, Falck JR, Aneja R, Aneja S, Parra A, Burns DJ, Ballas LM, Cantley LC. Activation of protein kinase C family members by the novel polyphosphoinositides PtdIns-3,4-P2 and PtdIns-3,4,5-P3. J Biol Chem. 1994;269:32358–32367. [Abstract] [Google Scholar]
- Tomiyama K, Makihara Y, Yamamoto H, O’Sullivan G, Nally RE, Tighe O, Kinsella A, Fienberg AA, Grandy DK, Sibley DR, Croke DT, Koshikawa N, Waddington JL. Disruption of orofacial movement topographies in congenic mutants with dopamine D5 but not D4 receptor or DARPP-32 transduction ‘knockout’ Eur Neuropsychopharmacol. 2006;16:437–445. [Abstract] [Google Scholar]
- Tong J, Ross BM, Sherwin AL, Kish SJ. Dopamine D1-stimulated adenylyl cyclase activity in cerebral cortex of autopsied human brain. Neurochem Int. 2001;39:117–125. [Abstract] [Google Scholar]
- Trivedi M, Narkar VA, Hussain T, Lokhandwala MF. Dopamine recruits D1A receptors to Na-K-ATPase-rich caveolar plasma membranes in rat renal proximal tubules. Am J Physiol Renal Physiol. 2004;287:F921–F931. [Abstract] [Google Scholar]
- Uberti MA, Hall RA, Minneman KP. Subtype-specific dimerization of alpha 1-adrenoceptors: effects on receptor expression and pharmacological properties. Mol Pharmacol. 2003;64:1379–1390. [Abstract] [Google Scholar]
- Undie AS. Biochemical evidence for D1 receptors not linked to adenylyl cyclase. In: Jenner P, Demirdamar R, editors. Dopamine Receptor Subtypes: From Basic Science to Clinical Application. IOS Press; Amsterdam: 1998. pp. 8–28. [Google Scholar]
- Undie AS. Relationship between dopamine agonist stimulation of inositol phosphate formation and cytidine diphosphate-diacylglycerol accumulation in brain slices. Brain Res. 1999;816:286–294. [Abstract] [Google Scholar]
- Undie AS. Modulation of cellular signaling pathways by antipsychotic drugs. In: Lidow MS, editor. Neurotransmitter receptors in actions of antipsychotic medications. CRC Press; Boca Raton: 2000. pp. 199–242. [Google Scholar]
- Undie AS. Dopaminergic modulation of phosphoinositide signaling cascades: implications for a new model of dopamine receptor function. DA2002 International Conference; Portland, OR. 2002. p. 33. [Google Scholar]
- Undie AS, Berki AC, Beardsley K. Dopaminergic behaviors and signal transduction mediated through adenylate cyclase and phospholipase C pathways. Neuropharmacology. 2000;39:75–87. [Abstract] [Google Scholar]
- Undie AS, Friedman E. Dopamine stimulates phosphoinositide metabolism in rat brain. Neurosci Res Commun. 1990a;6:69–75. [Google Scholar]
- Undie AS, Friedman E. Stimulation of a dopamine D1 receptor enhances inositol phosphates formation in rat brain. J Pharmacol Exp Ther. 1990b;253:987–992. [Abstract] [Google Scholar]
- Undie AS, Friedman E. Selective dopaminergic mechanism of dopamine and SKF38393 stimulation of inositol phosphate formation in rat brain. Eur J Pharmacol. 1992;226:297–302. [Abstract] [Google Scholar]
- Undie AS, Friedman E. Inhibition of dopamine agonist-induced phosphoinositide hydrolysis by concomitant stimulation of cyclic AMP formation in brain slices. J Neurochem. 1994;63:222–230. [Abstract] [Google Scholar]
- Undie AS, Weinstock J, Sarau HM, Friedman E. Evidence for a distinct D1-like dopamine receptor that couples to activation of phosphoinositide metabolism in brain. J Neurochem. 1994;62:2045–2048. [Abstract] [Google Scholar]
- Vanhaesebroeck B, Leevers SJ, Ahmadi K, Timms J, Katso R, Driscoll PC, Woscholski R, Parker PJ, Waterfield MD. Synthesis and function of 3-phosphorylated inositol lipids. Annu Rev Biochem. 2001;70:535–602. [Abstract] [Google Scholar]
- Venturini G, Stocchi F, Margotta V, Ruggieri S, Bravi D, Bellantuono P, Palladini G. A pharmacological study of dopaminergic receptors in planaria. Neuropharmacology. 1989;28:1377–1382. [Abstract] [Google Scholar]
- Vijayraghavan S, Wang M, Birnbaum SG, Williams GV, Arnsten AF. Inverted-U dopamine D1 receptor actions on prefrontal neurons engaged in working memory. Nat Neurosci. 2007;10:376–384. [Abstract] [Google Scholar]
- Vyas SJ, Eichberg J, Lokhandwala MF. Characterization of receptors involved in dopamine-induced activation of phospholipase-C in rat renal cortex. J Pharmacol Exp Ther. 1992a;260:134–139. [Abstract] [Google Scholar]
- Vyas SJ, Jadhav AL, Eichberg J, Lokhandwala MF. Dopamine receptor-mediated activation of phospholipase C is associated with natriuresis during high salt intake. Am J Physiol. 1992b;262:F494–F498. [Abstract] [Google Scholar]
- Waddington JL, Clifford JJ, McNamara FN, Tomiyama K, Koshikawa N, Croke DT. The psychopharmacology-molecular biology interface: exploring the behavioural roles of dopamine receptor subtypes using targeted gene deletion (‘knockout’) Prog Neuropsychopharmacol Biol Psychiatry. 2001;25:925–964. [Abstract] [Google Scholar]
- Waddington JL, Daly SA, Downes RP, Deveney AM, McCauley PG, O’Boyle KM. Behavioural pharmacology of ‘D-1-like’ dopamine receptors: further subtyping, new pharmacological probes and interactions with ‘D-2-like’ receptors. Prog Neuropsychopharmacol Biol Psychiatry. 1995;19:811–831. [Abstract] [Google Scholar]
- Wagner LE, Li WH, Yule DI. Phosphorylation of type-1 inositol 1,4,5-trisphosphate receptors by cyclic nucleotide-dependent protein kinases: a mutational analysis of the functionally important sites in the S2+ and S2− splice variants. J Biol Chem. 2003;278:45811–45817. [Abstract] [Google Scholar]
- Walaas SI, Aswad DW, Greengard P. A dopamine- and cyclic AMP-regulated phosphoprotein enriched in dopamine-innervated brain regions. Nature. 1983;301:69–71. [Abstract] [Google Scholar]
- Walaas SI, Greengard P. DARPP-32, a dopamine- and adenosine 3′:5′-monophosphate-regulated phosphoprotein enriched in dopamine-innervated brain regions. I. Regional and cellular distribution in the rat brain. J Neurosci. 1984;4:84–98. [Europe PMC free article] [Abstract] [Google Scholar]
- Walaas SI, Greengard P. Protein phosphorylation and neuronal function. Pharmacol Rev. 1991;43:299–349. [Abstract] [Google Scholar]
- Wallace MA, Claro E. Transmembrane signaling through phospholipase C in human cortical membranes. Neurochem Res. 1993;18:139–145. [Abstract] [Google Scholar]
- Waly M, Olteanu H, Banerjee R, Choi SW, Mason JB, Parker BS, Sukumar S, Shim S, Sharma A, Benzecry JM, Power-Charnitsky VA, Deth RC. Activation of methionine synthase by insulin-like growth factor-1 and dopamine: a target for neurodevelopmental toxins and thimerosal. Mol Psychiatry. 2004;9:358–370. [Abstract] [Google Scholar]
- Wang HY, Undie AS, Friedman E. Evidence for the coupling of Gq protein to D1-like dopamine sites in rat striatum: possible role in dopamine-mediated inositol phosphate formation. Mol Pharmacol. 1995;48:988–994. [Abstract] [Google Scholar]
- Watts VJ, Lawler CP, Gonzales AJ, zhou qy, Civelli O, Nichols DE, Mailman RB. Spare receptors and intrinsic activity: studies with D1 dopamine receptor agonists. Synapse. 1995;21:177–187. [Abstract] [Google Scholar]
- Weiner DM, Levey AI, Sunahara RK, Niznik HB, O’Dowd BF, Seeman P, Brann MR. D1 and D2 dopamine receptor mRNA in rat brain. Proc Natl Acad Sci U S A. 1991;88:1859–1863. [Europe PMC free article] [Abstract] [Google Scholar]
- Weinshank RL, Adham N, Macchi M, Olsen MA, Branchek TA, Hartig PR. Molecular cloning and characterization of a high affinity dopamine receptor (D1 beta) and its pseudogene. J Biol Chem. 1991;266:22427–22435. [Abstract] [Google Scholar]
- Welsh GI, Wilson C, Proud CG. GSK3: a SHAGGY frog story. Trends Cell Biol. 1996;6:274–279. [Abstract] [Google Scholar]
- White BH, Kimura K, Sidhu A. Inhibition of hormonally induced inositol trisphosphate production in Transfected GH4</ sup>C1 cells: A novel role for the D5 subtype of the dopamine receptor. Neuroendocrinology. 1999;69:209–216. [Abstract] [Google Scholar]
- Wilcox RA, Whitham EM, Liu C, Potter BV, Nahorski SR. Myo-inositol 1,3,4,5-tetrakisphosphate can independently mobilise intracellular calcium, via the inositol 1,4,5-trisphosphate receptor: studies with myo-inositol 1,4,5-trisphosphate-3-phosphorothioate and myo-inositol hexakisphosphate. FEBS Lett. 1993;336:267–271. [Abstract] [Google Scholar]
- Williams GV, Goldman-Rakic PS. Modulation of memory fields by dopamine D1 receptors in prefrontal cortex. Nature. 1995;376:572–575. [Abstract] [Google Scholar]
- Wojcikiewicz RJ, Luo SG. Phosphorylation of inositol 1,4,5-trisphosphate receptors by cAMP-dependent protein kinase. Type I, II, and III receptors are differentially susceptible to phosphorylation and are phosphorylated in intact cells. J Biol Chem. 1998;273:5670–5677. [Abstract] [Google Scholar]
- Yao LP, Li XX, Yu PY, Xu J, Asico LD, Jose PA. Dopamine D1 receptor and protein kinase C isoforms in spontaneously hypertensive rats. Hypertension. 1998;32:1049–1053. [Abstract] [Google Scholar]
- Young CE, Yang CR. Dopamine D1/D5 receptor modulates state-dependent switching of soma-dendritic Ca2+ potentials via differential protein kinase A and C activation in rat prefrontal cortical neurons. J Neurosci. 2004;24:8–23. [Europe PMC free article] [Abstract] [Google Scholar]
- Yu P, Yang Z, Jones JE, Wang Z, Owens SA, Mueller SC, Felder RA, Jose PA. D1 dopamine receptor signaling involves caveolin-2 in HEK-293 cells. Kidney Int. 2004;66:2167–2180. [Abstract] [Google Scholar]
- Yu Y, Wang JR, Sun PH, Guo Y, Zhang ZJ, Jin GZ, Zhen X. Neuroprotective effects of atypical D1 receptor agonist SKF83959 are mediated via D1 receptor-dependent inhibition of glycogen synthase kinase-3 beta and a receptor-independent anti-oxidative action. J Neurochem. 2008;104:946–956. [Abstract] [Google Scholar]
- Yung KK, Bolam JP, Smith AD, Hersch SM, Ciliax BJ, Levey AI. Immunocytochemical localization of D1 and D2 dopamine receptors in the basal ganglia of the rat: light and electron microscopy. Neuroscience. 1995;65:709–730. [Abstract] [Google Scholar]
- Zahrt J, Taylor JR, Mathew RG, Arnsten AF. Supranormal stimulation of D1 dopamine receptors in the rodent prefrontal cortex impairs spatial working memory performance. J Neurosci. 1997;17:8528–8535. [Europe PMC free article] [Abstract] [Google Scholar]
- Zanassi P, Paolillo M, Feliciello A, Avvedimento EV, Gallo V, Schinelli S. cAMP-dependent protein kinase induces cAMP-response element-binding protein phosphorylation via an intracellular calcium release/ERK-dependent pathway in striatal neurons. J Biol Chem. 2001;276:11487–11495. [Abstract] [Google Scholar]
- Zaworski PG, Alberts GL, Pregenzer JF, Im WB, Slightom JL, Gill GS. Efficient functional coupling of the human D3 dopamine receptor to G(o) subtype of G proteins in SH-SY5Y cells. Br J Pharmacol. 1999;128:1181–1188. [Europe PMC free article] [Abstract] [Google Scholar]
- Zeng C, Luo Y, Asico LD, Hopfer U, Eisner GM, Felder RA, Jose PA. Perturbation of D1 dopamine and AT1 receptor interaction in spontaneously hypertensive rats. Hypertension. 2003;42:787–792. [Abstract] [Google Scholar]
- Zhang L, Bai J, Undie AS, Bergson C, Lidow MS. D1 dopamine receptor regulation of the levels of the cell-cycle-controlling proteins, cyclin D, P27 and Raf-1, in cerebral cortical precursor cells is mediated through cAMP-independent pathways. Cereb Cortex. 2005;15:74–84. [Abstract] [Google Scholar]
- Zhang X, Zhou Z, Wang D, Li A, Yin Y, Gu X, Ding F, Zhen X, Zhou J. Activation of phosphatidylinositol-linked D1>-like receptor modulates FGF-2 expression in astrocytes via IP3-dependent Ca2+ signaling. J Neurosci. 2009;29:7766–7775. [Europe PMC free article] [Abstract] [Google Scholar]
- Zhen X, Goswami S, Friedman E. The role of the phosphatidyinositol-linked D1 dopamine receptor in the pharmacology of SKF83959. Pharmacol Biochem Behav. 2005;80:597–601. [Abstract] [Google Scholar]
- Zhen X, Torres C, Wang HY, Friedman E. Prenatal exposure to cocaine disrupts D1A dopamine receptor function via selective inhibition of protein phosphatase 1 pathway in rabbit frontal cortex. J Neurosci. 2001;21:9160–9167. [Europe PMC free article] [Abstract] [Google Scholar]
- Zhen X, Uryu K, Wang HY, Friedman E. D1 dopamine receptor agonists mediate activation of p38 mitogen-activated protein kinase and c-Jun amino-terminal kinase by a protein kinase A-dependent mechanism in SK-N-MC human neuroblastoma cells. Mol Pharmacol. 1998;54:453–458. [Abstract] [Google Scholar]
- Zheng S, Yu P, Zeng C, Wang Z, Yang Z, Andrews PM, Felder RA, Jose PA. Galpha12- and Galpha13-protein subunit linkage of D5 dopamine receptors in the nephron. Hypertension. 2003;41:604–610. [Abstract] [Google Scholar]
- Zhou Q-Y, Grandy DK, Thambi L, Kushner JA, Van Tol HH, Cone R, Pribnow D, Salon J, Bunzow JR, Civelli O. Cloning and expression of human and rat D1 dopamine receptors. Nature. 1990;347:76–80. [Abstract] [Google Scholar]
Full text links
Read article at publisher's site: https://doi.org/10.1016/j.pharmthera.2010.05.003
Read article for free, from open access legal sources, via Unpaywall:
https://europepmc.org/articles/pmc2939266?pdf=render
Citations & impact
Impact metrics
Citations of article over time
Alternative metrics

Discover the attention surrounding your research
https://www.altmetric.com/details/126697457
Article citations
Dopamine internalization via Uptake<sub>2</sub> and stimulation of intracellular D<sub>5</sub>-receptor-dependent calcium mobilization and CDP-diacylglycerol signaling.
Front Pharmacol, 15:1422998, 25 Oct 2024
Cited by: 0 articles | PMID: 39525629 | PMCID: PMC11543475
The Effect of Chronic Treatment with the Inhibitor of Phosphodiesterase 5 (PDE5), Sildenafil, in Combination with L-DOPA on Asymmetric Behavior and Monoamine Catabolism in the Striatum and Substantia Nigra of Unilaterally 6-OHDA-Lesioned Rats.
Molecules, 29(18):4318, 11 Sep 2024
Cited by: 0 articles | PMID: 39339313 | PMCID: PMC11434559
Rasd2 regulates depression-like behaviors via DRD2 neurons in the prelimbic cortex afferent to nucleus accumbens core circuit.
Mol Psychiatry, 03 Aug 2024
Cited by: 0 articles | PMID: 39097664
Dopamine enhances GABAA receptor-mediated current amplitude in a subset of intrinsically photosensitive retinal ganglion cells.
J Neurophysiol, 132(2):501-513, 03 Jul 2024
Cited by: 0 articles | PMID: 38958282
Modulation of D<sub>3</sub>R Splicing, Signaling, and Expression by D<sub>1</sub>R through PKA→PTB Phosphorylation.
Biomedicines, 12(1):206, 17 Jan 2024
Cited by: 0 articles | PMID: 38255311 | PMCID: PMC10813448
Go to all (69) article citations
Data
Data behind the article
This data has been text mined from the article, or deposited into data resources.
BioStudies: supplemental material and supporting data
Nucleotide Sequences (2)
- (1 citation) ENA - SKF83959
- (1 citation) ENA - SKF38393
Similar Articles
To arrive at the top five similar articles we use a word-weighted algorithm to compare words from the Title and Abstract of each citation.
High agonist-independent activity is a distinguishing feature of the dopamine D1B receptor subtype.
J Biol Chem, 269(45):27925-27931, 01 Nov 1994
Cited by: 125 articles | PMID: 7525564
Ethological resolution of behavioural topography and D1-like versus D2-like agonist responses in congenic D5 dopamine receptor mutants: identification of D5:D2-like interactions.
Synapse, 55(4):201-211, 01 Mar 2005
Cited by: 23 articles | PMID: 15668951
Dopamine receptor coupling to adenylyl cyclase in rat olfactory pathway: a combined pharmacological-radioautographic approach.
Neuroscience, 90(1):69-78, 01 Apr 1999
Cited by: 16 articles | PMID: 10188934
Dopamine-1 receptor G-protein coupling and the involvement of phospholipase A2 in dopamine-1 receptor mediated cellular signaling mechanisms in the proximal tubules of SHR.
Clin Exp Hypertens, 19(1-2):131-140, 01 Jan 1997
Cited by: 15 articles | PMID: 9028641
Review