Abstract
Free full text

Extracellular matrix, regional heterogeneity of the aorta, and aortic aneurysm
Abstract
Aortic aneurysm is an asymptomatic disease with dire outcomes if undiagnosed. Aortic aneurysm rupture is a significant cause of death worldwide. To date, surgical repair or endovascular repair (EVAR) is the only effective treatment for aortic aneurysm, as no pharmacological treatment has been found effective. Aortic aneurysm, a focal dilation of the aorta, can be formed in the thoracic (TAA) or the abdominal (AAA) region; however, our understanding as to what determines the site of aneurysm formation remains quite limited. The extracellular matrix (ECM) is the noncellular component of the aortic wall, that in addition to providing structural support, regulates bioavailability of an array of growth factors and cytokines, thereby influencing cell function and behavior that ultimately determine physiological or pathological remodeling of the aortic wall. Here, we provide an overview of the ECM proteins that have been reported to be involved in aortic aneurysm formation in humans or animal models, and the experimental models for TAA and AAA and the link to ECM manipulations. We also provide a comparative analysis, where data available, between TAA and AAA, and how aberrant ECM proteolysis versus disrupted synthesis may determine the site of aneurysm formation.
Introduction
Aortic aneurysm is morphologically defined as a focal dilation and structural degradation of the aorta, which can occur at different sites along the aortic tree. Pathologically, formation and progression of an aortic aneurysm is driven by dysregulated cellular and acellular events. While thorough reviews on the cellular mechanisms and current medical management of thoracic aortic aneurysm (TAA)1,2 and abdominal aortic aneurysm (AAA)2–4 have been published elsewhere, this review focuses on the role of the aortic extracellular matrix (ECM) and the proteases and their inhibitors that maintain the integrity and homeostasis of the ECM in the pathogenesis of TAA or AAA in humans and animal models.
Aortic wall structure and the ECM
The aorta is the largest conduit artery in the body. Owing to its extraordinary ability to expand and recoil, the aorta also serves as a reservoir that transforms the highly pressured and pulsatile heart output into a flow of moderate fluctuations. The composition and three-dimensional organization of its ECM is critical for optimal physiological functions of the aorta. The aortic wall comprises an intricate arrangement of endothelial cells (ECs), smooth muscle cells (SMCs), fibroblasts (FBs), and ECM proteins in three layers: tunica intima, tunica media, and tunica adventitia (Fig. (Fig.1).1). The tunica intima consists of a single layer of ECs that lines the lumen of the blood vessel, and is anchored to the underlying basement membrane, a highly specialized ECM network consisting primarily of laminin, collagen type IV, fibronectin, perlecan, and heparan sulfate proteoglycans5. This basement membrane also plays a pivotal role in signaling events that regulate EC migration, invasion, proliferation, and survival6. The basement membrane together with the internal elastic lamina (IEL) serves as an interface between the tunica intima and tunica media. IEL is a thin sheet of elastic fiber with a variable number of fenestrations that facilitate diffusion of molecules through the tunica intima to the tunica media7. The tunica media consists of concentric elastic lamellae with circumferentially oriented SMCs. This layer is rich in ECM proteins, such as proteoglycans (PGs), glycoproteins, glycosaminoglycans (GAGs), as well as collagen. The intermittent layers of elastic fibers and SMCs in this layer provide the compliance and recoil properties of the aortic wall8. The medial layer of the aortic wall is the most variable of the tunics among different arteries. The differences in ECM composition in this layer account for the main variations in the function and properties of the arterial tree. The external elastic lamina (EEL) forms a boundary between the tunica media and tunica adventitia, the outermost layer of the aortic wall that is rich in collagen, FBs, and elastic fiber, and provides the tensile strength of the aortic wall. This collagen-rich tunica also harbors innervations, lymphatics, and the vasa vasorum, which are responsible for the high susceptibility of the adventitial layer to vascular inflammation.
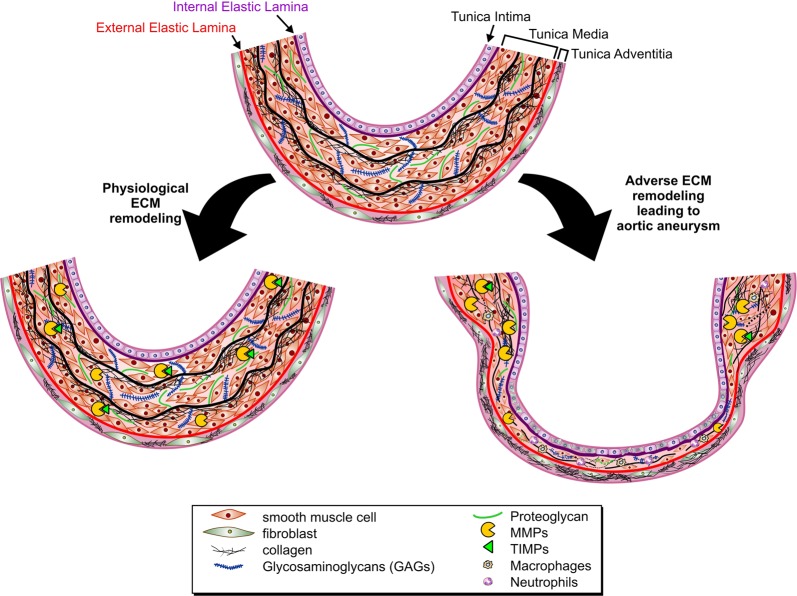
Cross section of the aortic wall and its remodeling during physiological versus pathological remodeling. The tunica media is separated from the tunica intima by the internal elastic lamina, and from the tunica adventitia by the external elastic lamina. Intermittent layers of elastic fiber and smooth muscle cells make up the tunica media, while this region is also rich in proteoglycans and glycoproteins. During physiological remodeling, degradation of ECM proteins by MMPs (and other proteases) is balanced by newly synthesized ECM proteins, while tissue inhibitors of proteases (TIMPs) keep the proteolytic activity of MMPs under check. Excess ECM degradation, or impaired ECM renewal synthesis, along with smooth muscle cell death, lead to adverse ECM remodeling as observed in aortic aneurysm
The ECM is a key constituent of the vascular wall. SMCs and the FBs in the aortic wall synthesize and organize a complex ECM structure that defines the passive mechanical behavior of the large, elastic arteries such as the aorta. Arterial ECM is primarily composed of elastin, collagen, proteoglycans (PGs), and glycoproteins. Elastic fibers and fibrillar collagen, comprising ~50% of the dry weight of larger arteries9, are the predominant ECM components in the aortic wall and almost entirely define the mechanical properties of the aorta. Elastic fibers provide the expandability and recoil properties, while fibrillar collagens (predominantly collagen type I and type III) are responsible for the tensile strength of the aortic wall to withstand the high pressure of blood pumped by the heart10. In addition to their mechanical properties of the aortic wall, elastic fibers and fibrillar collagens can directly interact with various integrins and other proteins to modulate the adhesion, proliferation, and migration of SMCs11–14. GAGs consist of linear chains of repeating disaccharides and are highly negatively charged, which allow them to sequester water. Hydrated GAGs occupy a large volume of the extracellular space and cushion-compressive forces on cells. Among the four primary classes of GAGs, hyaluronan, chondroitin sulfate, heparan sulfate, and keratan sulfate, the first three are present in the aortic wall15,16. PGs are proteins with covalently attached GAG chains that provide mechanical support and mediate signaling responses for cells in the aortic wall. Versican, which is rich in chondroitin sulfate, is among the principal proteoglycans present in the aorta17. Among small leucine-rich proteoglycans (SLRP), biglycan and decorin are reported to promote collagen fibrinogenesis in the aorta18,19. Thrombospondins, tenascins, and fibronectin are also common glycoproteins present in the aorta20,21.
Regional heterogeneity of the aortic wall
Morphology, structural composition, and mechanical properties of the aortic wall vary in the ascending and along the descending regions22 to ensure optimal mechanical operation23. The tunica intima accounts for <1% of the total wall thickness and remains uniform along the aorta, whereas the thickness of tunica adventitia is negligible in the thoracic aorta but increases considerably along the abdominal aorta24. The medial layer exhibits the greatest regional variations and diversitise along the aorta. The thickness of tunica media and the aortic lumen size steadily decrease from the ascending to the descending aorta, such that the wall thickness-to-lumen diameter ratio remains constant throughout the aorta. In the thoracic aorta, elastin occupies a higher proportion of the tunica media compared with that in the abdominal aorta. The amount of elastin decreases with distance from the heart, as less pulse dampening is required in the distal aorta. Meanwhile, the amount of collagen, which provides strength and limits stretch at high pressure, remains approximately constant with distance from the heart25. Therefore, the elastin-to-collagen ratio is lower in the abdominal compared with the thoracic aorta. In addition, there is a linear relationship between the number of lamellar units and the tensional forces within the aortic wall, and the number of lamellar units decreases from the thoracic to the abdominal aorta10.
Physiological remodeling of the ECM
The ECM composition and integrity are key determinants of the physical characteristics of the aortic wall. Vascular ECM undergoes continuous physiological remodeling, whereby the existing ECM proteins are proteolytically degraded to be replaced by newly synthesized proteins. Several protease families are involved in this process. Matrix metalloproteinases (MMPs) are the most explored ECM-degrading proteinases26, while other metalloproteinases such as ADAMs (a disintegrin and metalloproteinases), ADAM-TSs (ADAMs with thrombospondin motifs), or serine/cysteine proteases, including cathepsins and granzymes, can also contribute to this process.
MMPs are a family of zinc-containing calcium-dependent endopeptidases that are best known for their ECM-degrading function, although they also contribute to a number of other cellular events26. To date, 23 human MMPs have been identified, which are classified into six groups based on their substrate specificities: collagenase, gelatinase, stromelysin, matrilysin, membrane-type MMPs, and others. MMPs are mostly secreted as inactive zymogens and are activated by proteolytic removal of their N-terminal pro-domain. The proteolytic activity of MMPs is tightly regulated in vivo by their endogenous inhibitors, tissue inhibitor of metalloproteinases (TIMPs)27,28. Other MMP inhibitors, such as α2-macroglobulin, inhibit MMPs in the plasma and liquid phase by irreversibly removing them through endocytosis29. The four members of the TIMP family (TIMP-1, −2, −3, and −4) can collectively inhibit all MMPs and a number of ADAMs and ADAM-TSs30 through a covalent bond between their N terminus and the catalytic domain of the proteinase in a 1:1 stoichiometric ratio. In addition to inhibiting metalloproteinases, TIMPs play a number of MMP-independent functions31–34. Sustained balance in proteolytic turnover of the ECM proteins is critical in maintaining the structural and functional integrity of the aortic wall. Cathepsins, a family of serine, cysteine, and aspartyl proteases are lysosomal proteases that also possess extracellular functions and can cleave multiple ECM components, including fibronectin, laminin, and elastin, and a number of non-ECM components35. Granzymes are serine proteases that contribute to aortic remodeling and have been the subject of a recent review36.
Aortic aneurysm
Aortic aneurysm is characterized by a localized irreversible dilatation of the aortic lumen by ≥50% of its original diameter. The dilatation is induced by aberrant and adverse remodeling of the aortic wall, including endothelial damage in the intima, SMC loss and ECM degradation in the media, and ECM degradation in the adventitia. Aortic aneurysm affects all three layers of the blood vessel, while any dilation of the aorta that is limited to the outer wall is considered a false aneurysm or pseudo aneurysm37. Aortic aneurysm is asymptomatic, but can progress, gradually leading to aortic dissection or rupture, and as such is associated with critical morbidity and mortality in the aging population and accounts for 1–2% of total death in developed countries38. Although TAA and AAA display similar physical appearances, they each demonstrate distinct etiologies, pathological features, epidemiology, and risk factors.
Abdominal aortic aneurysm (AAA)
AAA represents dilation of the aorta in any portion of the infra-diaphragmatic aorta, including the most common site of AAA formation, the infrarenal aorta. Depending on the degree of dilatation, AAA severity is classified as small (<55mm) or large (≥55
mm). In the latter case, the patient will be considered eligible for surgical repair39. AAA is the 12–15th leading cause of death in the aged population, mostly males, over the age of 65 in many developed countries4. Almost 1–2% of all 65-year-old males have AAA, whereas 0.5% of women at 70 years of age are affected with AAA40,41. The overall mortality due to AAA rupture has been reported to be 65–85%38. However, AAA-related mortalities in both male and female populations are declining in developed countries42, with the United States and the United Kingdom showing the fastest decline in AAA mortalities in male (6.7–6.2% per year) and female (3.9–4% per year) populations. This was found to closely correlate with the decline in smoking prevalence in these countries. On the other hand, in many European countries, including Romania and Hungary, AAA mortalities have increased by 1.7–2.7% for males and 1–3.5% for females annually42.
AAA is a complex multifactorial disease that is affected by both environmental and genetic factors. AAA incidences depend on age and sex, with a higher prevalence in males over the age of 55 compared with females of the same age range (6:1)43. However, AAA appears more detrimental in females, as they usually experience a higher risk of a small aneurysm rupture and less eligibility for standard surgical repair, because of the more complicated aneurysm morphology44,45. Other risk factors include smoking, a family history of aneurysm46, the presence of other diseases such as coronary heart disease, hyperlipidemia and atherosclerosis, hypertension, as well as acute or chronic infection4,38. Tobacco smokers have 3–4-fold greater risk of developing AAA than nonsmokers47,48. The link between hypertension and AAA is paradoxical, since hypertension has been shown to increase the risk of AAA by 30–40%; however, antihypertensive medication further increased the risk by 70–80%47. More recently, a randomized placebo-controlled trial reported that antihypertensive medications did not impact AAA growth, despite effective lowering of BP49. Interestingly, patients with diabetes mellitus have shown decreased prevalence or progression of AAA50, which could be linked to the effects of antidiabetic medications. Hypercholesterolemia, increased body mass index, lack of exercise, and cardiovascular diseases were found to be associative factors for higher incidences of AAAs in Swedish male population51. Although atherosclerosis shows a close association with AAA, multiple studies have demonstrated the lack of a causal role for atherosclerosis in the initiation of AAA52–54.
Thoracic aortic aneurysm (TAA)
TAA represents dilation of one or more aortic segments above the diaphragm, including the aortic root, ascending aorta, aortic arch, and descending aorta. Approximately 60% of TAA occurs in the aortic root or the ascending aorta, 10% in the aortic arch, 40% in the descending aorta, and 10% in the thoraco-abdominal aorta55. About 13% of patients having aortic aneurysm develop multiple aneurysms, and ~20% of them can have a large TAA along with an AAA56. The prevalence of TAA is much less than that of AAA57,58, but the prognosis of TAA is more dire, and the rate of rupture-associated mortality is 2–3 times higher than that for AAA patients. While AAA is more commonly found in elders, TAA can occur at a young age due to the strong hereditary influence59.
Similar to AAA, no causal relationship has been found between atherosclerosis and TAA60, and it is relatively less associated with ascending TAA55. TAA often results from genetic disorders, and is now referred to as syndromic or familial TAA. In a study of 135 non-syndromic TAA patients without Marfan syndrome (MFS), almost 19% of the patients were found to have a family history of TAA and developed the disease in relatively a younger age61. Moreover, patients with a family history of TAA were found to have a faster aneurysm growth rate (0.22cm/year) compared to patients with sporadic TAA (0.03
cm/year) or patients with MFS (0.10
cm/year)61. The specific genetic mutations associated with TAA are discussed later in this review. TAA has also been linked to inflammation and inflammatory diseases. Syphilis was once considered as one of the most common causes of ascending TAA55, and although extremely rare nowadays, recent cases of syphilis aortitis and TAA have been reported62. Aortic arteritis, which includes Takayasu’s arteritis and giant-cell arteritis, refers to a group of chronic inflammatory diseases of unknown etiologies, and is associated with a high prevalence of TAA63,64.
Recent clinical studies with large scale populations have identified fluoroquinolones, a commonly prescribed class of antibiotics as a risk factor for aortic aneurysm65,66. Fluoroquinolone usage elevated the risk of aortic aneurysm or dissection by 2–3 folds compared with the nontreatment group67,68, and by 2-fold compared with other antibiotics such as amoxicillin69. Fluoroquinolones are believed to affect the synthesis and structural integrity of collagen in the aortic wall68, or their chelating properties could affect collagen cross-linking and metalloprotease activities70,71. In a mouse model of AAA, ciprofloxacin (a fluoroquinolone) treatment significantly reduced lysyl oxidase (LOX) expression and activity, and increased MMP expression and activity72. However, the exact biological mechanism of action for this class of antibiotics requires further investigation.
Experimental models of aortic aneurysm
Understanding the cellular and molecular event during the initiation, maturation, and rupture of human aortic aneurysm holds the key to identifying molecule(s) that are critical in the pathogenesis of this disease. However, aneurysmal aorta specimens from patients are only available at the advanced stages of the disease and therefore are not suitable for identifying the initiating factors. As such, a number of experimental models of aortic aneurysm have been developed and utilized on various genetically modified mice to understand the molecular mechanism(s) underlying aortic aneurysm (Table (Table1).1). Most of these experimental models share some of the features of human aortic aneurysm, although they all fall short of exactly mimicking the full clinical characteristics of this disease in humans3,73. Nevertheless, these models have helped provide insight into understanding the disease pathogenesis and can be utilized for the possible discovery of novel therapies for aortic aneurysm.
Table 1
The impact of genetic alterations of ECM-related genes on aortic aneurysm
Mouse strain | Aneurysm model used | Phenotype | Ref. |
---|---|---|---|
Mmp2−/− | CaCl2-induced TAA, AAA | Protection against AAA, TAA | 83,108 |
Ang II infusion | Exacerbated aortic dilation, TAA | 83 | |
Apoe−/−/Mmp3−/− | Cholesterol-rich diet | Reduced aneurysm formation | 110 |
Mmp9−/− | Intraluminal elastase-induced AAA | Protection against AAA | 84 |
Mmp12−/− | CaCl2-induced AAA | Protection against AAA | 97 |
Wild-type/Mmp14−/− bone marrow chimera | CaCl2-induced AAA | Reduced formation of AAA | 109 |
Adamts1 transgenic/Apoe−/− | Cholesterol-rich diet and Ang II infusion | No changes observed | 115 |
Adamts4−/− | High-fat diet and Ang II infusion | Reduced aneurysm, dissection, and aortic rupture | 98 |
Adamts5Δcat (Truncated catalytic domain) | Ang II infusion | Increased dilatation of the ascending aorta | 114 |
SMC- or EC-specific Adam17−/− | Adventitial elastase-induced TAA | Protection against TAA | 88 |
SMC-specific Adam17−/− | Ang II![]() ![]() | Protection against TAA | 116 |
Apoe−/−/Cats−/− | Ang II infusion | Protection against AAA | 119 |
Catk−/− | Intraluminal elastase-induced AAA | Reduced AAA | 120 |
Catl−/− | Intraluminal elastase-induced AAA | Reduced AAA | 121 |
Apoe−/−/Plat−/− | High cholesterol diet | No changes observed | 125 |
Apoe−/−/Plau−/− | High cholesterol diet | Protected against media destruction and aneurysm | 125 |
Plg−/− | CaCl2-induced AAA | Reduced AAA | 126 |
SMC-specific Pai1 overexpression | Aortic xenograft | Prevented AAA formation | 127 |
Apoe−/−/Gzmb−/− | Ang II-induced AAA | Protection against AAA | 129 |
Timp1−/− | Intraluminal elastase-induced AAA | Enhanced aneurysm | 131 |
Timp1−/− | CaCl2-induced TAA | Enhanced aneurysm | 132 |
SMC-specific Timp1 overexpression | Aortic xenograft AAA | Prevented AAA degeneration and rupture | 133 |
Timp2−/− | CaCl2-induced AAA | Protection against AAA | 137 |
Timp3−/− | Ang II infusion | Enhanced AAA | 82 |
Timp3−/−/Mmp2−/− | Ang II infusion | Exacerbated AAA | 82 |
Eln−/− | Spontaneous | Perinatal death | 143 |
Eln+/- | Spontaneous | Supravalvular aortic stenosis | 143 |
Fbln4−/− | Spontaneous | Perinatal death due to abolished elastogenesis | 180 |
SMC-specific Fbln4−/− | Spontaneous | Spontaneous ascending and thoracic aortic aneurysm | 181 |
Fbn1 mutant | Spontaneous | Arteriopathy, aneurysm, and dissection | 182 |
Col1a1 Δ/ Δ (first intron deleted) | Spontaneous | Age-dependent aortic dissection and rupture | 145 |
Col3a1+/− | Spontaneous | Sudden death due to TAA, dissection, and rupture | 146 |
LoxMut/Mut | Spontaneous | Spontaneous death after birth due to aortic rupture | 149 |
Bgn−/− | Spontaneous | Spontaneous aortic dissection and rupture | 18 |
Apoe−/−/Dcn overexpression | Ang II infusion | Reduced AAA and rupture | 19 |
SMC-specific Fbln4−/−/Thbs1−/− | Spontaneous | Attenuated TAA compared with Fbln4−/− | 21 |
Thbs4−/− | Ang II infusion | Attenuated TAA | 159 |
Fbln fibulin, Fbn fibrillin, Cat cathepsin, Bgn biglycan, Dcn decorin, Plat total plasminogen activator, Plau urokinase-plasminogen activator, Plg plasminogen, Pai plasminogen activator inhibitor, Gzmb granzyme B, Mmp matrix metalloproteinase, Apoe apolipoprotein E, Adam a disintegrin and metalloproteinase, Adamts ADAMs with thrombospondin motifs, Timp tissue inhibitor of metalloproteinases, Eln elastin, Col collagen, Lox lysyl oxidase, SMC smooth muscle cell, EC endothelial cell, Ang II angiotensin II
(i) Hyperlipidemic model
The hyperlipidemic model of aortic aneurysm utilizes subcutaneous infusion of a high dose of angiotensin II (Ang II, 1.5mg/kg/d) in hyperlipidemic mice, namely mice lacking apolipoprotein E (Apoe−/−) or low-density lipoprotein receptor (Ldlr−/−) receiving a high-fat diet73,74. Long-term high-fat diet (HFD) feeding induces aortic inflammation and atherosclerosis in these mice75. This model is associated with medial phagocyte accumulation, medial dissection, elastic network degradation, and inflammatory cell infiltration76. The hyperlipidemic models of AAA share some features of obesity-induced vascular inflammation, atherosclerosis, and aortic aneurysm in humans.
(ii) Angiotensin II infusion model
Subcutaneous infusion of Ang II, in the absence of hyperlipidemia, is commonly used in mice and rats to induce cardiovascular remodeling77. This relatively easy and minimally invasive surgical model mimics some features of human aortic aneurysm, including ECM remodeling, SMC activation, and vascular inflammation73. When used in combination with β-aminopropionitrile (BAPN), an inhibitor of collagen cross-linking enzyme LOX, Ang II infusion can result in the formation of TAA or AAA, or aortic dissection78,79. Simultaneous use of BAPN and a TGFβ inhibitor has also been reported to induce AAA in mice80,81. These aneurysm models display an aggressive development of intraluminal thrombus, elastin fragmentation, and influx of a range of inflammatory cells to the aortic wall81. Being a time-dependent progressive model, Ang II-induced aneurysm can be utilized for preventive and/or therapeutic studies. Intriguingly, Ang II infusion alone, in the absence of BAPN or hyperlipidemia, triggered AAA in Timp3−/− mice82, and TAA in Mmp2−/− mice83. As discussed in later sections, these two genetic models exhibit the importance of ECM-regulatory proteins in sustaining the homeostasis of aortic wall structure and the regional susceptibility of the aorta to aneurysm formation.
(iii) Intraluminal elastase model
Intraluminal elastase perfusion model introduces porcine pancreatic elastase (PPE, 0.45 U/ml) into the infrarenal aortic lumen (at 100mmHg, 5
min), which results in AAA formation within 2 weeks84. This model combines the mechanical strain with the enzymatic degradation of the elastic network in the aortic wall by PPE to induce injury, inflammation, and ultimately aneurysm85. This model is technically challenging as it requires the infrarenal aorta to be surgically separated from the inferior vena cava, and the lumbar and the juxtarenal arteries and the aortic bifurcation need to be temporarily ligated before the infrarenal aorta is punctured and infused with PPE3. As such, this model can only be used to induce AAA and not TAA since the close proximity of the thoracic aorta to the lungs and the heart drastically reduces the margin of error.
(iv) Periadventitial elastase model
Periadventitial elastase model utilizes PPE to trigger degradation of the aortic elastic network by exposing the adventitia to elastase86. In this model, PPE (15 or 30U/mL) is directly applied onto the adventitial surface of the aorta for 5 to 10
min to induce AAA86 or TAA87,88, respectively. This model is markedly less invasive than the intraluminal elastase model as it does not require aortic puncture nor pressure-induced mechanical damage. In addition, the adventitial elastase model can be used to induce AAA86 or TAA87,88. Although intraluminal and adventitial elastase models result in morphologically distinct aortic aneurysms in mice and thus represent specific subtypes of this disease in humans89, these aneurysms do not display thrombus, atherosclerosis, or rupture, which are classical features of human aortic aneurysm. A recent study showed that a combination of oral administration of BAPN and periadventitial elastase application induced a chronic, advanced-stage AAA with persistent growth, thrombus, and spontaneous rupture80. This represents a promising animal model to better understand the pathogenesis of AAA and to test promising therapeutic compounds.
(v) Calcium chloride or calcium phosphate model
This model requires application of concentrated calcium chloride (CaCl2)90 or calcium phosphate (CaCl2 and PBS)85 solutions onto the adventitial layer of the aorta to induce calcification, elastin degradation, and subsequently aneurysm formation in 4–8 weeks. This model is site-specific and can be used to induce AAA and TAA83. Of note, CaCl2-induced TAA can be associated with a high operative mortality rate due to pulmonary complications upon exposure to CaCl2. In addition, the severity of aortic aneurysm in this model can be relatively mild.
(vi) Decellularized aortic xenograft model
This model involves surgical interspecies transplantation of a decellularized aorta segment, for instance from guinea pig to rat91,92. The underlying principle of this model is to differentiate the impact of the cellular component versus the ECM. In the absence of the histocompatibility complex and protease inhibitors that are synthesized by the cellular compartment of the aorta, the decellularized aortic xenograft becomes the target of immune reaction and proteolytic degradation of the ECM and progressive aortic dilation92,93. Similar to human AAA, this model develops intraluminal thrombus and aortic aneurysm and rupture92.
(vii) Large animal models
Large animal models of TAA and AAA are less diverse and less prevalently used compared to the rodent models, and have been proven useful for preclinical testing of endovascular devices or surgical procedures, rather than aneurysm pathogenesis. Intraluminal PPE perfusion has also been used in dogs, which, similar to the rodent model, leads to disruption of the medial elastic lamellae and aneurysm formation94. However, this model requires high concentrations of elastase and aneurysm formation is inconsistent. To avoid these issues, intra-aortic elastase and collagenase perfusion combined with aortic balloon angioplasty has been used95. In a pig model of TAA, intra-adventitial injection of collagenase with periadventitial application of crystalline CaCl2 resulted in elastic lamellar degradation, decreased collagen content, and TAA formation after 3 weeks96.
In summary, although each experimental model represents select characteristics of aortic aneurysm in the context of associated comorbidity(ies), and therefore can be useful in understanding the pathology of this disease. However, the interpretation of the findings should be exclusively based on the model used since contradictory results can be observed when the same animals are subjected to different models. For example, Mmp12-deficiency in mice suppresses CaCl2-induced AAA formation97, but does not affect elastase-induced AAA formation84.
ECM-regulatory proteins in aortic aneurysm
Aortic aneurysm involves dramatic changes in the structural components of the vasculature, due to SMC dysfunction, imbalance between proteinases and their inhibitors, and abnormal synthesis, accumulation, or degradation of ECM proteins3. Among different classes of proteases, metalloproteinases (MMPs, ADAMs, and ADAM-TSs)83,88,98,99, and cathepsins100,101 are important factors for pathogenesis of aneurysm, because of their involvement in cleavage of different ECM and non-ECM proteins. The availability of genetically modified mouse models has paved the way to understanding the role of the ECM and its remodeling, the importance of protease and their inhibitors, and the contribution of different proteoglycans and glycoproteins in aortic aneurysm.
Matrix metalloproteinases
Multiple MMPs contribute to physiological aortic remodeling and their increased expression are well documented in both human and mouse aortic aneurysms102. Under physiological conditions, vascular SMCs (VSMC) are the major source of MMPs production in the aorta, which is amplified under inflammatory conditions leading to augmented expression of MMPs103. Inflammatory cells, such as neutrophils and macrophages, also contribute to MMP production which further promote ECM degradation and aneurysm formation. Neutrophils can produce MMP8 and MMP9104,105, whereas macrophages can secrete a larger number of MMPs, including MMP1, MMP3, MMP7, MMP9, and MMP12106. These MMPs can cleave collagen (MMP1, MMP2, MMP9, MMP13, and MT1-MMP107), elastin (MMP2, MMP9, and MMP12), proteoglycans and basement membrane proteins, leading to the degradation of aortic ECM and aneurysm formation103.
Studies on MMP-deficient mice have provided us with important insight into the role of specific MMPs in different models of aortic aneurysm. Mmp2-deficient mice showed reduced aortic aneurysm formations in CaCl2-induced TAA and AAA models83,108. However, in response to Ang II infusion, Mmp2-deficient mice developed severe TAA but not AAA83. The absence of MMP9 in mice attenuated AAA formations84; however, the protection was reversed by introducing wild-type macrophages in Mmp9−/− mice, but not in Mmp2−/− mice, highlighting the role of macrophage MMP9 and stromal MMP2 in the pathogenesis of AAA108. Even in the absence of MMP2 or MMP9, MT1-MMP can be a key regulatory factor to induce aortic aneurysm by promoting macrophage-dependent elastolytic activity in CaCl2-induced AAA109. Apoe−/−/Mmp3−/− mice showed reduced aortic aneurysm formation despite persistence of atherosclerotic plaques compared with Apoe−/− mice110. Loss of MMP12, also known as macrophage metalloelastase, attenuated aneurysm formation in CaCl2-induced AAA model97.
Although there is significant evidence that MMPs are upregulated in AAA and TAA, and MMP-deficiency often results in protection against aneurysm formation, MMP inhibition may not be an effective approach in treating aortic aneurysm. Consistently, broad-spectrum MMP inhibitors, such as doxycycline, were reported to have promising effects in treating small AAAs111. However, recent randomized trials reported no changes in AAA growth or in reducing the need for AAA repair or time to repair in patients receiving doxycycline112.
Disintegrin and metalloproteinases (ADAMs, ADAM-TSs)
A number of ADAM-TSs, including ADAM-TS4, ADAM-TS5, and ADAM-TS7, have been reported to be involved in TAA in humans and in mouse models98,113,114. Mice expressing truncated ADAM-TS5 (lacking the catalytic domain) showed increased aortic dilation in Ang II-induced TAA compared to wild-type mice114. ADAM-TS4 deficiency reduced aortic dilation, aneurysm formation, dissection and aortic rupture in a mouse model of sporadic aortic aneurysm and dissection (AAD) induced by HFD and Ang II infusion98. However, ADAM-TS1 overexpressing Apoe−/− mice subjected to HFD and Ang II infusion did not show elevated aneurysm response compared to Apoe−/− mice115.
Compared with ADAM-TSs, the role of ADAMs in aortic aneurysm is less explored. The most studied ADAM, ADAM17, was found to be critically involved in aortic aneurysm development in both humans and mice88. SMC- or EC-specific deletion of ADAM17 protected the aorta from elastase-induced TAA formation88, whereas ADAM17 deficiency in SMCs provided protection against AAA triggered by Ang II+
BAPN treatment116. ADAM10 is an important regulator for vascular remodelling and inhibition of ADAM10 mRNA with miR-103a prevented AAA formation in Apoe−/− mice following HFD and Ang II treatment117.
Cathepsins
Cathepsins are a group of serine, aspartic, and cysteine proteases, which contribute to vascular remodelling. Several cathepsins, including cathepsins A, D, S, K, and L are reported to be involved or upregulated in humans aortic aneurysmal tissues100,101,118. Cathepsin K and S are potent elastases that are produced by SMCs and macrophages in the aorta under inflammatory milieus. Deficiency of cathepsin S attenuated Ang II-induced AAA formation in Apoe−/− mice119. Cathepsin K has been reported to contribute to CD4+ T-cell proliferation and SMC apoptosis since its deficiency attenuated the AAA in the intraluminal elastase infusion model in mice120. Cathepsin L knockout mice exhibited reduced lesions, in vitro macrophage and T-cell transmigration, and angiogenic responses upon intraluminal elastase-induced AAA development121.
Other proteases
There are several other proteases that are important in aortic aneurysm formation. Chymase and plasminogen activators are serine proteases that are involved in activation of other proteases, including MMPs. Chymases, secreted mostly by mast cells, activate MMP9 in human AAAs122. In addition to converting plasminogen into plasmin, plasminogen activators can directly degrade ECM components, activate collagenases, and degranulate neutrophils to release MMPs and elastases123,124. Deficiency of urokinase-plasminogen activator, but not tissue-plasminogen activator suppressed aortic aneurysm formation in hypercholesteremic Apoe−/− mice125. Plasminogen deficiency also attenuated CaCl2-induced AAA by targeting macrophage migration and MMP9 activation126. Alternatively, local overexpression of plasminogen activator inhibitor-1 (PAI-1) prevented aneurysm development and rupture in mice127. Granzyme B is a proapoptotic serine protease that is abundantly expressed in advanced human atherosclerotic lesions and may contribute to plaque instability128. Granzyme B contributes to AAA through an extracellular, perforin-independent mechanism involving ECM cleavage. The absence of Granzyme B decreased AAA formation and increase survival of Apoe−/− mice upon Ang II infusion129.
Tissue inhibitors of metalloproteinase (TIMP)
TIMPs regulate the proteolytic activities in the vascular wall by directly binding to and inhibiting different groups of metalloproteinases, including MMPs, ADAMs, and ADAM-TSs. Although there is a high degree of structural homology among the four members of the TIMP family, they exhibit varying substrate affinities, inhibitory efficiencies, and differential responses to pathological stimuli130. TIMP1 can inhibit most members of the MMP family except the membrane-type MMPs (MT-MMPs) and MMP19, and few ADAMs, such as ADAM10130. TIMP1 expression is reduced in aneurysmal tissues, and the absence of TIMP1 resulted in severe aneurysm in the intraluminal elastase-perfused AAA131 and CaCl2-induced TAA mouse models132. Local overexpression of TIMP1 prevented aortic aneurysm degeneration and rupture in a rat model133. TIMP2 which acts as an inhibitor for many MMPs134 and ADAM12135, also plays an important role in MMP2 activation in coordination with MT1-MMP136. TIMP2 deficiency resulted in protection against CaCl2-induced AAA in mice137, perhaps by inhibiting MMP2 activation. TIMP3 has the broadest range of substrates among TIMPs which includes most of the MMPs, many ADAMs (ADAM −10, −12, −17, −19, and −33) and ADAMTSs (ADAMTS-1, −2, −4, and −5)28. Moreover, TIMP3 is distinct from other TIMPs in being ECM-bound138. Increased expression of TIMP3 in aneurysmal aortic tissue suggests its protective role against the disease139, as the absence of TIMP3 promoted aortic dilation and aneurysm in Ang II-induced (non-hyperlipidemic) AAA82. TIMP4 can inhibit a number of MMPs and ADAM28140. Interestingly, the N-terminal domain of TIMP4, but not the full-length protein, can inhibit ADAM17141. The role of TIMP4 in aortic aneurysm is less explored. Increased TIMP4 expression has been reported in hyperhomocysteinemia-associated aortic aneurysm in humans and mice142.
ECM proteins that are central to aneurysm formation
Elastin
Elastin is one of the principle ECM molecules synthesized by SMCs in the aorta in response to mechanical stress or pressure, and is formed as a complex of elastin protein assembled on a microfibril platform. Elastic fibers provide the aortic wall with a unique ability to expand and recoil that is essential for optimal blood perfusion throughout the body. Fragmentation of elastic fibers is a common feature of aortic aneurysm independent from the initiating factors, in both humans and animal models. Deletion of the elastin gene (Eln) in mice resulted in an uncontrolled proliferation of SMCs and perinatal death due to high blood pressure and tortuous, stenotic arteries. While elastin haploinsufficiency (Eln+/−) improved survival, it resulted in supravalvular aortic stenosis143.
Collagen
Fibrillar collagens type I and type III account for 80–90% of the total collagen present in the aorta, while collagens type IV, V, VI, and VII represent the remaining fraction of collagens. Collagen turnover is critical for vessel wall repair and regeneration. In addition to its contribution to the vascular structure and tensile strength, it can regulate cell proliferation through interacting with integrins14. In humans, higher levels of collagens type I/III and collagen cross-linking are reported in aneurysmal aortas144, as increased collagen can enhance arterial stiffness and susceptibility to dissection and rupture. On the other hand, decreased collagen content and cross-linking can weaken the aortic wall, leading to aneurysm formation and/or aortic dissection144. The disparity in collagen content might reflect different phases of aortic remodeling, with fibrosis occurring at the late phase of inflammation during vessel repair. This also highlights the importance of sustaining a balance in collagen content for optimal aortic structure and function. Deletion of the first intron of Col1α1 gene resulted in an age-dependent aortic dissection and rupture due to gradual decrease in collagen content145. Haploinsufficiency of Col3α1 caused aortic dissection in mice and a pathology similar to the vascular-type Ehlers–Danlos syndrome (VEDS)146. Appropriate cross-linking of collagen monomers by LOX is critical for collagen fibril formation147, as inactivation of LOX, through mutations or gene deletion, can lead to TAA, cardiovascular dysfunction, and perinatal death in mice148–150.
Proteoglycans/glycoproteins
Proteoglycans and glycoproteins comprise the non-fibrillar fraction of the ECM, which fills the extracellular space that is not occupied by the fibrillar ECM, and interacts with various molecules (growth factors, cytokines, etc.) to mediate their sequestration within the ECM. Several proteoglycans and glycoproteins have been reported to be upregulated during aortic inflammation and aneurysms151. The proteoglycans in the aortic wall mainly include large proteoglycans, such as versicans and aggrecans, or SLRP such as decorin, lumican, biglycan, etc152. These proteins are also involved in developing the pericellular matrix and promoting proliferation and migration of VSMC153. Versican is present in the intimal and medial layers of the aorta and is mostly expressed at the external part of the medial layer during vascular inflammation17,154. TAA and dissections are reported to have massive aggrecan and versican accumulation155; however, the levels of cleaved versican (V0 isoform) are decreased17. Biglycan is expressed throughout the aortic wall and regulates collagen fibrillogenesis156. Biglycan knockout mice develop spontaneous aortic dissection and rupture18. Similar to biglycan, decorin promotes collagen assembly and can be cleaved by Granzyme B, which has been associated with aortic aneurysm formation in mice128. Moreover, recombinant decorin fusion protein expression in mice attenuates Ang II-induced AAA formation and rupture19.
Thrombospondins, a family of secreted glycoproteins with antiangiogenic functions, are highly expressed in the aortic wall under inflammatory conditions. Thrombospondin-1 is reported to negatively regulate cell adhesion, migration, proliferation, and angiogenesis157. SMC-specific deletion of thrombospondin-1 attenuates TAA formation by restoring the disruption of the elastic lamina-SMC connections and preserving the mechano-transduction in mice and humans21. Thrombospondin-4 regulates vascular inflammation and atherogenesis158, and its deficiency can lead to aortic aneurysm formation following 3 weeks of Ang II infusion159. Fibronectin is a prevalent glycoprotein in the aortic wall, and its expression has been reported to be elevated in aneurysmal aorta from patients with bicuspid aortic valve and tricuspid aortic valve stenosis160. Recent studies have reported that impaired splicing of fibronectin is associated with TAA formation161. Tenascins are glycoproteins highly expressed in the aorta. Loss of tenascin C led to AAD in a combined CaCl2 and Ang II infusion model in mice, which was linked to enhanced inflammation, impaired TGFβ signaling, and collagen synthesis162.
Aortic aneurysm associated with mutations in ECM-regulatory genes in humans
Over the last few decades, clinical studies have revealed a growing connection between mutations in ECM proteins and aortic aneurysm in humans (Table (Table2).2). Many of these mutations are well known, as they underlie the heritable syndromic diseases such as MFS, Loeys–Dietz syndrome (LDS), and Ehlers–Danlos syndrome (EDS); others are mostly responsible for non-syndromic aneurysm-related disorders163,164. MFS is one of the most extensively studied autosomal dominant disorder of the connective tissues. MFS affects the skeletal, ocular, and cardiovascular systems and is often associated with the development of TAA165. The incidence frequency of MFS is around 1:5,000 and it is implicated in 3–5% of all aortic dissections166. MFS is the result of mutations of the fibrilin-1 gene (Fbn1). Fibrillin-1 is a large, ECM glycoprotein that serves as a structural component of calcium-binding microfibrils167 that is central to elastic fiber assembly. Mutation of Fbn1 impairs elastic fiber structure and promotes dilatation of the aortic root and the ascending aorta in the affected patients, causing aortic dissection and hemorrhage which can lead to sudden deaths165,167. Fibrillin-2 (Fbn2) is mostly associated with aortic development, and plays a major role during early morphogenesis in directing elastic fiber assembly and is found preferentially in elastic tissues, such as the cartilage, the tunica medial layer of the aorta and along the bronchial tree168. Mutations in Fbn2 cause congenital contractual arachnodactyly, an autosomal dominant syndrome associated with aortic root dilation and aneurysm169. Fibrillin proteins exhibit marked structural homology to latent TGFβ binding protein, and are believed to influence TGFβ signaling in MFS163,170. However, the importance of TGFβ signaling in aortic aneurysm is independently recognized in another human aortopathy, LDS. LDS is an autosomal dominant disorder characterized by aggressive aortic root and TAAs where multiple family members are responsible for different types of LDS171. All five LDS variations (LDS1-5) are characterized by respective mutations of the following genes, Tgfbr1, Tgfbr2, Smad3, Tgfb2, and Tgfb3. The mutations present in Tgfbr1 and Tgfbr2 are principally missense and mostly occur in the kinase domains of the receptors172.
Table 2
ECM protein mutations associated with hereditary aortic aneurysm in humans
ECM gene | Identified genetic alterations | Syndrome/phenotype | Ref. |
---|---|---|---|
Col1α1 | Missense mutations | Osteogenesis imperfecta; Ehlers–Danlos syndrome type 7A | 175 |
Col1α2 | Missense mutations | Osteogenesis imperfecta; Ehlers–Danlos syndrome type 7B | 175,183 |
Col3α1 | Multi-exon deletions | Ehlers–Danlos syndrome, type 4 | 173,184 |
Col4α1 | Mutations in exon 24, exon 25 | Hereditary angiopathy, nephropathy, and aneurysms | 177 |
Col4α5 | Nonsense mutations | X-linked Alport syndrome; TAA, AAA | 178 |
Eln | Multiple point mutations | Supravalvular aortic stenosis; ascending aortic aneurysm and dissection | 179,185 |
Fbn1 | Missense mutations | Marfan syndrome; TAA | 186 |
Fbn2 | Mutations of intron 32, resulting in missplicing of exon 32 | Cutis laxa with aneurysm; TAA | 169 |
Fbln4 | Heterozygous mutations | Cutis laxa with aneurysm | 187 |
Lox1 | Loss-of-function missense mutation | TAA and dissection | 149,150 |
Smad3 | Mutations at the MH2 domain | Loeys–Dietz syndrome type 3 | 188 |
Tgfβ2 | Heterozygous mutations or microdeletions | Loeys–Dietz syndrome type 4 | 189 |
Tgfβr1 | Heterozygous mutations | Loeys–Dietz syndrome type 1 | 190 |
Tgfβr2 | Heterozygous mutations | Loeys–Dietz syndrome type 2 | 190,191 |
VEDS is an autosomal dominant trait, which results from heterogenous mutation of type III procollagen gene (Col3α1) leading to frequent arterial dissections with TAA. VEDS is one of the most prevalent vasculopathies with an incidence rate of 1:5,000–20,000 in developed countries173. In the aorta, collagen supports and maintains the tensile strength and stiffness of the aorta for which collagen maturation and cross-linking are important. For proper assembly of collagen alpha mono-trimers into triple-helix collagen, it is important to have the repetitive presence of Gly–X–Y amino acid sequences in all three alpha chains. In VEDS, however, the glycine is replaced by other amino acids, which disrupts the formation of collagen alpha trimers and subsequently, intact collagen fibrils174. Individuals with VEDS are at risk of arterial rupture, aneurysm and dissection, uterine rupture during pregnancy, and gastrointestinal perforation or rupture which could cause sudden death164. Mutations in collagen genes can also induce aortic dysfunction. Mutations of Col1α1 and Col1α2 are reported in patients with osteogenesis imperfecta with rare aortic aneurysm175. However, Col1α1 mutation has been associated with dissections in medium-sized arteries and Col1α2 mutation with borderline aortic root enlargement with regurgitation in patients175,176. Collagen IV is a major component of the basement membrane in various tissues including the aorta, and mutation of Col4α1 gene has been linked to hereditary angiopathies, including nephropathy and aneurysm177. Mutation of Col4α5 is associated with X-linked Alport syndrome and ascending aortic and AAA and dissection178. A missense mutation in the Lox gene has been linked to TAA and aortic dissection because of insufficient elastin and collagen cross-linking in the aortic wall149.
Elastin is a key structural component in cardiovascular development, vascular elasticity and structural integrity of the aorta. Decreased elastin in humans is associated with development of an autosomal dominant disease, supravalvular aortic stenosis (SVAS). SVAS results from loss-of-function mutations in the elastin gene and is associated with aortic stenosis, hypertension, and cardiac failure179.
Conclusion/future prospects
In conclusion, aortic aneurysm remains a serious health concern as its rupture can cause significant morbidity and mortality. It is well acknowledged that TAA is a distinct disease from AAA and should be treated as such. Research to date suggests that given the regional heterogeneity of the aortic structure and ECM, events that disrupt ECM synthesis or ECM protein assembly could underlie the formation of aneurysm in the thoracic area, whereas factors that trigger enhanced proteolytic degradation of the ECM contribute to AAA formation. This is consistent with the notion that genetic disorders that disrupt collagen or elastin production (or assembly) are associated mostly with TAA and to a smaller extent with AAA. While studies on genetically modified mice have revealed a number of potential molecular and cellular mechanisms that may contribute to TAA or AAA, the lack of availability of an effective pharmacological treatment indicates that we may not yet have the full picture of all the factors that contribute to aortic aneurysm. Identifying the initiating factors in aneurysm formation is key in developing a treatment strategy, since at later stages, to repair the severe damage on the aortic wall if possible, would require effective replenishment of ECM-producing cells and ensuring optimal assembly and organization of the newly synthesized ECM proteins.
Acknowledgements
This work was supported by a project grant from Canadian Institute for Health Research (CIHR) to Z.K. M.H. is supported by Chinese Scholarship Council (CSC), Z.K. is a member of the Royal Society of Canada, and a Canada Research Chair scientist (Tier 1) in Cardiovascular Extracellular Matrix.
Authors’ contributions
S.J. and Z.K. planned the outline of the article. S.J. and M.H. prepared the first draft, M.S. performed critical editing of the article. S.J. and Z.K. prepared the tables and the figure, revised, and finalized the article.
Footnotes
Publisher’s note: Springer Nature remains neutral with regard to jurisdictional claims in published maps and institutional affiliations.
References
Articles from Experimental & Molecular Medicine are provided here courtesy of Korean Society for Biochemistry and Molecular Biology
Full text links
Read article at publisher's site: https://doi.org/10.1038/s12276-019-0286-3
Read article for free, from open access legal sources, via Unpaywall:
https://www.nature.com/articles/s12276-019-0286-3.pdf
Citations & impact
Impact metrics
Article citations
CardioGraph: a platform to study variations associated with familiar cardiopathies.
BMC Med Inform Decis Mak, 23(suppl 3):303, 21 Oct 2024
Cited by: 0 articles | PMID: 39434095 | PMCID: PMC11494761
The Causal Relationship between Immune-Mediated Inflammatory Diseases and Aortic Aneurysm: A Bidirectional Two-Sample Mendelian Randomization Study.
J Immunol Res, 2024:2474118, 09 Oct 2024
Cited by: 0 articles | PMID: 39421304 | PMCID: PMC11483648
Targeting the smooth muscle cell Keap1-Nrf2-GSDMD-pyroptosis axis by cryptotanshinone prevents abdominal aortic aneurysm formation.
Theranostics, 14(17):6516-6542, 07 Oct 2024
Cited by: 0 articles | PMID: 39479449 | PMCID: PMC11519792
Ultrasound Probe Pressure Affects Aortic Wall Stiffness: A Patient-Specific Computational Study in Abdominal Aortic Aneurysms.
Ann Biomed Eng, 04 Sep 2024
Cited by: 0 articles | PMID: 39230788
Wall shear stress measured with 4D flow CMR correlates with biomarkers of inflammation and collagen synthesis in mild-to-moderate ascending aortic dilation and tricuspid aortic valves.
Eur Heart J Cardiovasc Imaging, 25(10):1384-1393, 01 Sep 2024
Cited by: 0 articles | PMID: 38748858 | PMCID: PMC11441033
Go to all (87) article citations
Similar Articles
To arrive at the top five similar articles we use a word-weighted algorithm to compare words from the Title and Abstract of each citation.
Pathophysiology of thoracic aortic aneurysm (TAA): is it not one uniform aorta? Role of embryologic origin.
Prog Cardiovasc Dis, 56(1):68-73, 15 May 2013
Cited by: 47 articles | PMID: 23993239 | PMCID: PMC3759819
Review Free full text in Europe PMC
Endovascular repair of abdominal aortic aneurysm: an evidence-based analysis.
Ont Health Technol Assess Ser, 2(1):1-46, 01 Mar 2002
Cited by: 3 articles | PMID: 23074438 | PMCID: PMC3387737
Loss of TIMP3, but not TIMP4, exacerbates thoracic and abdominal aortic aneurysm.
J Mol Cell Cardiol, 184:61-74, 14 Oct 2023
Cited by: 1 article | PMID: 37844423
Pathogenic mechanisms and the potential of drug therapies for aortic aneurysm.
Am J Physiol Heart Circ Physiol, 318(3):H652-H670, 21 Feb 2020
Cited by: 28 articles | PMID: 32083977 | PMCID: PMC7099451
Review Free full text in Europe PMC
Funding
Funders who supported this work.